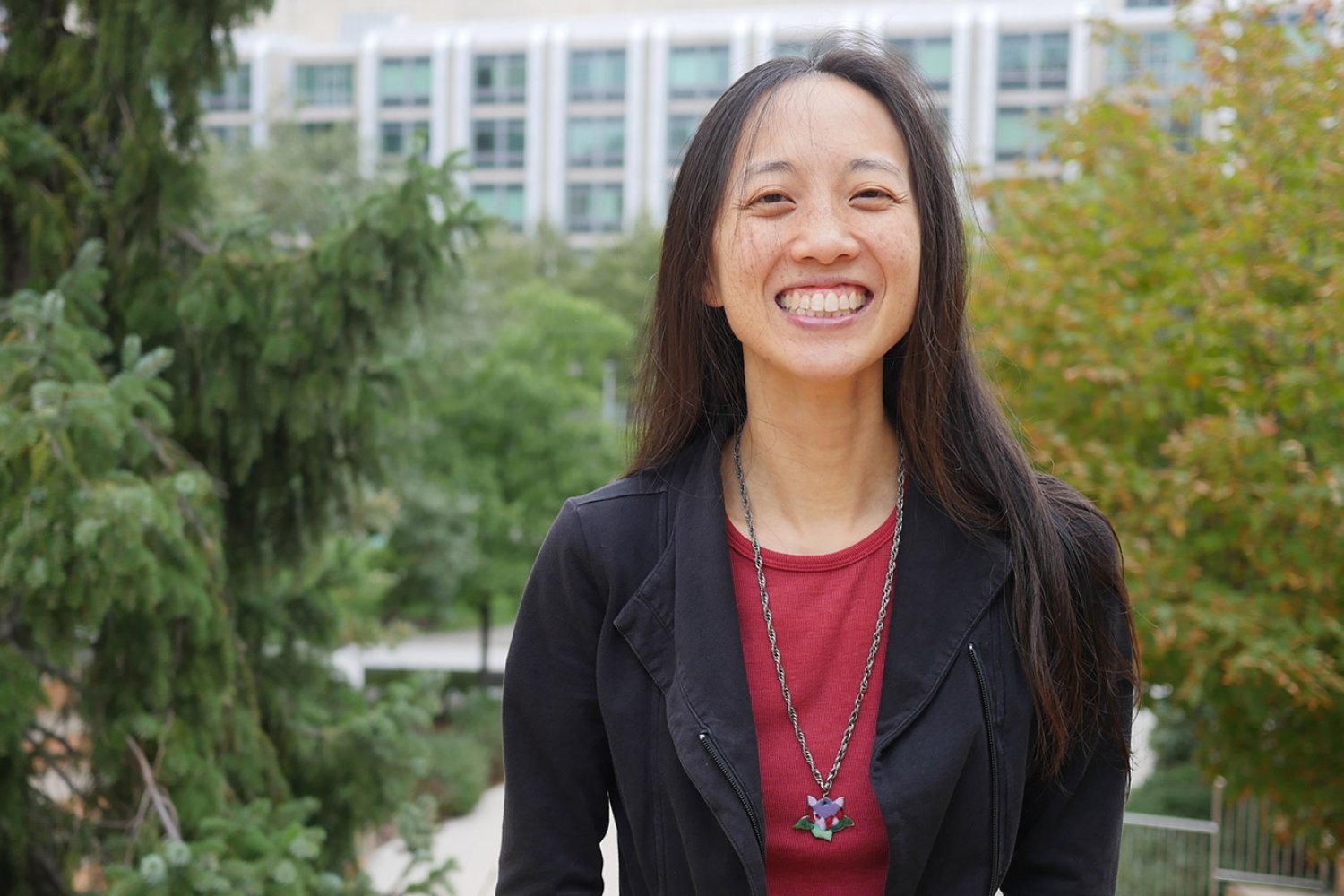
Senior Technical Instructor Vanessa Cheung ’02 brings the energy, experience, and excitement needed to educate students in the biology teaching lab.
Samantha Edelen | Department of Biology
April 29, 2025
For more than 30 years, Course 7 (Biology) students have descended to the expansive, windowless basement of Building 68 to learn practical skills that are the centerpiece of undergraduate biology education at the Institute. The lines of benches and cabinets of supplies that make up the underground MIT Biology Teaching Lab could easily feel dark and isolated.
In the corner of this room, however, sits Senior Technical Instructor Vanessa Cheung ’02, who manages to make the space seem sunny and communal.
“We joke that we could rig up a system of mirrors to get just enough daylight to bounce down from the stairwell,” Cheung says with a laugh. “It is a basement, but I am very lucky to have this teaching lab space. It is huge and has everything we need.”
This optimism and gratitude fostered by Cheung is critical, as MIT undergrad students enrolled in classes 7.002 (Fundamentals of Experimental Molecular Biology) and 7.003 (Applied Molecular Biology Laboratory) spend four-hour blocks in the lab each week, learning the foundations of laboratory technique and theory for biological research from Cheung and her colleagues.
Running toward science education
Cheung’s love for biology can be traced back to her high school cross country and track coach, who also served as her second-year biology teacher. The sport and the fundamental biological processes she was learning about in the classroom were, in fact, closely intertwined.
“He told us about how things like ATP [adenosine triphosphate] and the energy cycle would affect our running,” she says. “Being able to see that connection really helped my interest in the subject.”
That inspiration carried her through a move from her hometown of Pittsburgh, Pennsylvania, to Cambridge, Massachusetts, to pursue an undergraduate degree at MIT, and through her thesis work to earn a PhD in genetics at Harvard Medical School. She didn’t leave running behind either: To this day, she can often be found on the Charles River Esplanade, training for her next marathon.
She discovered her love of teaching during her PhD program. She enjoyed guiding students so much that she spent an extra semester as a teaching assistant, outside of the one required for her program.
“I love research, but I also really love telling people about research,” Cheung says.
Cheung herself describes lab instruction as the “best of both worlds,” enabling her to pursue her love of teaching while spending every day at the bench, doing experiments. She emphasizes for students the importance of being able not just to do the hands-on technical lab work, but also to understand the theory behind it.
“The students can tend to get hung up on the physical doing of things — they are really concerned when their experiments don’t work,” she says. “We focus on teaching students how to think about being in a lab — how to design an experiment and how to analyze the data.”
Although her talent for teaching and passion for science led her to the role, Cheung doesn’t hesitate to identify the students as her favorite part of the job.
“It sounds cheesy, but they really do keep the job very exciting,” she says.
Using mind and hand in the lab
Cheung is the type of person who lights up when describing how much she “loves working with yeast.”
“I always tell the students that maybe no one cares about yeast except me and like three other people in the world, but it is a model organism that we can use to apply what we learn to humans,” Cheung explains.
Though mastering basic lab skills can make hands-on laboratory courses feel “a bit cookbook,” Cheung is able to get the students excited with her enthusiasm and clever curriculum design.
“The students like things where they can get their own unique results, and things where they have a little bit of freedom to design their own experiments,” she says. So, the lab curriculum incorporates opportunities for students to do things like identify their own unique yeast mutants and design their own questions to test in a chemical engineering module.
Part of what makes theory as critical as technique is that new tools and discoveries are made frequently in biology, especially at MIT. For example, there has been a shift from a focus on RNAi to CRISPR as a popular lab technique in recent years, and Cheung muses that CRISPR itself may be overshadowed within only a few more years — keeping students learning at the cutting edge of biology is always on Cheung’s mind.
“Vanessa is the heart, soul, and mind of the biology lab courses here at MIT, embodying ‘mens et manus’ [‘mind and hand’],” says technical lab instructor and Biology Teaching Lab Manager Anthony Fuccione.
Support for all students
Cheung’s ability to mentor and guide students earned her a School of Science Dean’s Education and Advising Award in 2012, but her focus isn’t solely on MIT undergraduate students.
In fact, according to Cheung, the earlier students can be exposed to science, the better. In addition to her regular duties, Cheung also designs curriculum and teaches in the LEAH Knox Scholars Program. The two-year program provides lab experience and mentorship for low-income Boston- and Cambridge-area high school students.
Paloma Sanchez-Jauregui, outreach programs coordinator who works with Cheung on the program, says Cheung has a standout “growth mindset” that students really appreciate.
“Vanessa teaches students that challenges — like unexpected PCR results — are part of the learning process,” Sanchez-Jauregui says. “Students feel comfortable approaching her for help troubleshooting experiments or exploring new topics.”
Cheung’s colleagues report that they admire not only her talents, but also her focus on supporting those around her. Technical Instructor and colleague Eric Chu says Cheung “offers a lot of help to me and others, including those outside of the department, but does not expect reciprocity.”
Professor of biology and co-director of the Department of Biology undergraduate program Adam Martin says he “rarely has to worry about what is going on in the teaching lab.” According to Martin, Cheung is ”flexible, hard-working, dedicated, and resilient, all while being kind and supportive to our students. She is a joy to work with.”