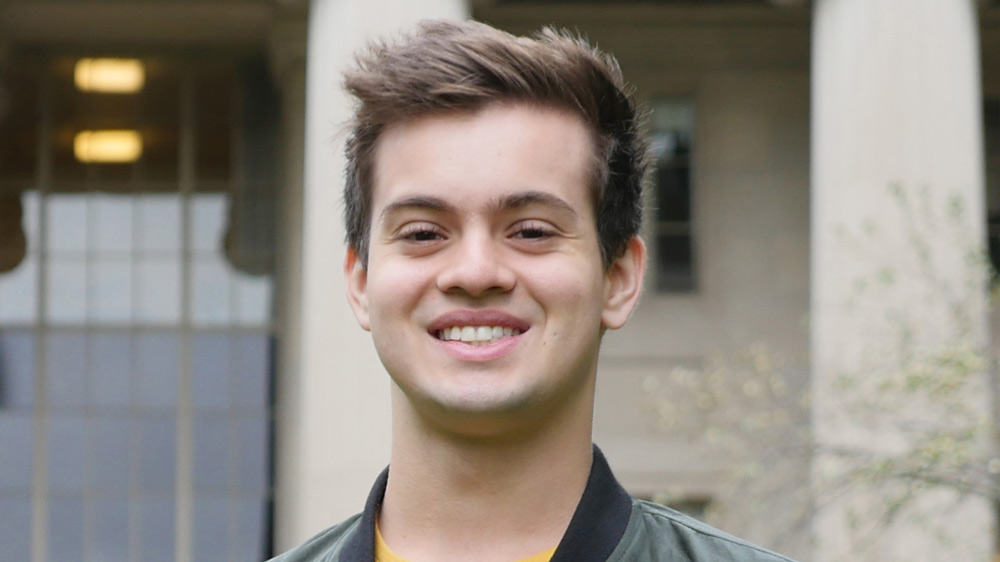
Undergraduate Camilo Espinosa grew up with a love for math, before developing a second passion for immunology at MIT
Raleigh McElvery
November 10, 2017
Biology by Numbers
Undergraduate Camilo Espinosa grew up with a love for math, before developing a second passion for immunology at MIT
Raleigh McElvery
Undergraduate Camilo Espinosa, now in his senior year, tackles biological problems with the mindset of a mathematician. That’s because he initially approached the STEM fields (science, technology, engineering and mathematics) starting with the “M” and ending with the “S” — developing an appetite for math before realizing a second love for biology.
Every six months, beginning his first year of middle school, Espinosa would venture from his home on the north coast of Colombia to the nation’s capital. There, for several weeks, he would do nothing but math.
“These were math olympiads — basically a combination of math camp and competitions,” he explains. “That was the first real community I had outside my school.”
But he didn’t just glean formulas and analytical strategies from those competitions; it was his olympiad team that first introduced him to MIT. In Colombia, he explains, students must select a major almost immediately upon entering university, and are offered limited electives. MIT came to represent “academic freedom” for Espinosa, who, despite his avid and early love of math, intended to explore multiple academic avenues before limiting himself to just one.
Now, as a math and chemistry-biology double major with a concentration in philosophy, he says MIT has enabled him to pursue his many academic interests, as well as his non-academic ones. He has served as an active member of not one but four dance teams, as well as president of his fraternity. He also helped establish a channel of communication between the International Students Office and the Department of Biology, to streamline the work authorization process for international students.
He was drawn to biology, he explains, because he prefers learning processes over basic facts. “I don’t care much for memorization, but I do care about the underlying reasons for why and how things function,” he says. “I think that mindset stems from my dad.”
Espinosa’s father is an OB/GYN specializing in female oncology, who initially helped to popularize laparoscopic surgery techniques in Colombia. Often, he sees patients at a discounted price or for free if they could not afford his services.
“At the end of the day, he is just trying to help people,” Espinosa explains. “He taught me the way I think about the world. He’s the reason I do what I do, and why I’m so oriented towards the life sciences.”
Espinosa’s siblings are studying to become doctors and veterinarians, and he himself is intrigued by the possibilities of using our body’s innate defense mechanisms to treat diseases like cancer.
His foray into the field of immunology began with antibodies — special ones taken from furry, gawky alpacas — so tiny and versatile that they can be employed for all manner of imaging, therapeutic, and diagnostic techniques. These “single domain” antibodies were a popular area of interest in Hidde Ploegh’s lab (formerly a Professor of Biology at the Whitehead Institute for Biomedical Research), where Espinosa began mid-way through October of his freshman year. Using these single-domain antibodies, the Ploegh lab had developed a treatment for melanoma in mice, and Espinosa worked to pinpoint antibodies directed against melanoma in humans.
The summer between his sophomore and junior years, Espinosa began a separate project that eventually evolved into his thesis. He honed in on one tiny antibody, known as A4, which binds to a particular protein expressed on the surface of red blood cells, and has the potential to thwart the immune response by activating a process known as “tolerance.”
Our body’s immune system is programmed to discern self from non-self, targeting foreign entities for destruction. It does so in two distinct steps. First, it creates an army of cells, that together express antibodies tailored to combat virtually every possible substance, both self and non-self. The immune system is then primed to spring into action whenever it senses something foreign, amplifying those cells that express antibodies against it. However, the body would also attack itself if not for the second step in this process: tolerance. The immune system essentially deletes the cells expressing antibodies against itself, and in doing so learns to “tolerate” its own proteins.
For example, when red blood cells die of old age (and many do every day), this triggers tolerance to the various protein components that constitute those cells — preventing related antibodies from being created.
Previous work has shown that binding a foreign protein to red blood cells triggers tolerance for that specific protein, despite being “non-self.” This could have implications for therapies to treat conditions like hemophilia, Espinosa explains, which require injections of proteins to reinstate the body’s blood-clotting abilities.
In a large proportion of patients, the immune system responds and attacks these proteins as foreign, rendering the treatment useless and barring the patients from receiving it again in the future. However, Espinosa proposes, if he could couple A4 to the treatment protein, then A4 would link the protein to the red blood cells and initiate tolerance to it. Since the body can no longer create antibodies against the treatment proteins, the therapy can run its course. In other words, the body would now see the injected proteins as self.
After months of methodical experiments, A4 didn’t appear to disguise proteins as self in the way Espinosa had initially hoped, although it did reduce the immune response triggered by the protein injection, if he staggered the protein and antibody infusions in the proper manner.
“So maybe A4 doesn’t work as a camouflage per se, but rather as a suppressor of certain immune responses,” he says. “So the results didn’t turn out exactly as we expected, but it is still a step in the right direction.”
He ultimately submitted his thesis to MIT’s Ilona Karmel Writing Prizes, earning second place in the technical writing category.
Last summer, Espinosa explored a different side of basic research — the corporate side — during an internship at the biotechnology company Genentech. There, he investigated the pathways by which uncontrolled cell death leads to sepsis in patients.
As he wraps up his senior year and begins applying to graduate programs, Espinosa reflects on his transition from student to instructor, having served as a teaching assistant in a number of biology courses during the past three years. “As someone who arrived at MIT with a weak foundation in biology, almost everything I’ve learned since was because someone taught it to me, and taught it to me well,” he says. “I feel honored to be able to pass it on.”