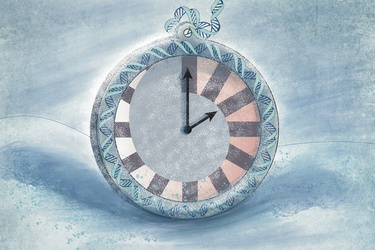
New research from the Hrvatin Lab recently published in Nature Aging indicates that inducing a hibernation-like state in mice slows down epigenetic changes that accompany aging.
Shafaq Zia | Whitehead Institute
March 7, 2025
Surviving extreme conditions in nature is no easy feat. Many species of mammals rely on special adaptations called daily torpor and hibernation to endure periods of scarcity. These states of dormancy are marked by a significant drop in body temperature, low metabolic activity, and reduced food intake—all of which help the animal conserve energy until conditions become favorable again.
The lab of Whitehead Institute Member Siniša Hrvatin studies daily torpor, which lasts several hours, and its longer counterpart, hibernation, in order to understand their effects on tissue damage, disease progression, and aging. In their latest study, published in Nature Aging on March 7, first author Lorna Jayne, Hrvatin, and colleagues show that inducing a prolonged torpor-like state in mice slows down epigenetic changes that accompany aging.
“Aging is a complex phenomenon that we’re just starting to unravel,” says Hrvatin, who is also an assistant professor of biology at Massachusetts Institute of Technology. “Although the full relationship between torpor and aging remains unclear, our findings point to decreased body temperature as the central driver of this anti-aging effect.”
Tampering with the biological clock
Aging is a universal process, but scientists have long struggled to find a reliable metric for measuring it. Traditional clocks fall short because biological age doesn’t always align with chronology—cells and tissues in different organisms age at varying rates.
To solve this dilemma, scientists have turned to studying molecular processes that are common to aging across many species. This, in the past decade, has led to the development of epigenetic clocks, new computational tools that can estimate an organism’s age by analyzing the accumulation of epigenetic marks in cells over time.
Think of epigenetic marks as tiny chemical tags that cling either to the DNA itself or to the proteins, called histones, around which the DNA is wrapped. Histones act like spools, allowing long strands of DNA to coil around them, much like thread around a bobbin. When epigenetic tags are added to histones, they can compact the DNA, preventing genetic information from being read, or loosen it, making the information more accessible. When epigenetic tags attach directly to DNA, they can alter how the proteins that “read” a gene bind to the DNA.
While it’s unclear if epigenetic marks are a cause or consequence of aging, this much is evident: these marks change over an organism’s lifespan, altering how genes are turned on or off, without modifying the underlying DNA sequence. These changes have enabled researchers to track the biological age of individual cells and tissues using dedicated epigenetic clocks.
In nature, states of stasis like hibernation and daily torpor help animals survive by conserving energy and avoiding predators. But now, emerging research in marmots and bats hints that hibernation may also slow down epigenetic aging, prompting researchers to explore whether there’s a deeper connection between prolonged bouts of torpor and longevity.
However, investigating this link has been challenging, as the mechanisms that trigger, regulate, and sustain torpor remain largely unknown. In 2020, Hrvatin and colleagues made a breakthrough by identifying neurons in a specific region of the mouse hypothalamus, known as the avMLPA, which act as core regulators of torpor.
“This is when we realized that we could leverage this system to induce torpor and explore mechanistically how the state of torpor might have beneficial effects on aging,” says Jayne. “You can imagine how difficult it is to study this in natural hibernators because of accessibility and the lack of tools to manipulate them in sophisticated ways.”
The age-old mystery
The researchers began by injecting adeno-associated virus in mice, a gene delivery vehicle that enables scientists to introduce new genetic material into target cells. They employed this technology to instruct neurons in the mice’s avMLPA region to produce a special receptor called Gq-DREADD, which does not respond to the brain’s natural signals but can be chemically activated by a drug. When the researchers administered this drug to the mice, it bound to the Gq-DREADD receptors, activating the torpor-regulating neurons and triggering a drop in the animals’ body temperature.
However, to investigate the effects of torpor on longevity, the researchers needed to maintain these mice in a torpor-like state for days to weeks. To achieve this, the mice were continuously administered the drug through drinking water.
The mice were kept in a torpor-like state with periodic bouts of arousal for a total of nine months. The researchers measured the blood epigenetic age of these mice at the 3-, 6-, and 9-month marks using the mammalian blood epigenetic clock. By the 9-month mark, the torpor-like state had reduced blood epigenetic aging in these mice by approximately 37%, making them biologically three months younger than their control counterparts.
To further assess the effects of torpor on aging, the group evaluated these mice using the mouse clinical frailty index, which includes measurements like tail stiffening, gait, and spinal deformity that are commonly associated with aging. As expected, mice in the torpor-like state had a lower frailty index compared to the controls.
With the anti-aging effects of the torpor-like state established, the researchers sought to understand how each of the key factors underlying torpor—decreased body temperature, low metabolic activity, and reduced food intake—contributed to longevity.
To isolate the effects of reduced metabolic rate, the researchers induced a torpor-like state in mice, while maintaining the animal’s normal body temperature. After three months, the blood epigenetic age of these mice was similar to that of the control group, suggesting that low metabolic rate alone does not slow down epigenetic aging.
Next, Hrvatin and colleagues isolated the impact of low caloric intake on blood epigenetic aging by restricting the food intake of mice in the torpor-like state, while maintaining their normal body temperature. After three months, these mice were a similar blood epigenetic age as the control group.
When both low metabolic rate and reduced food intake were combined, the mice still exhibited higher blood epigenetic aging after three months compared to mice in the torpor state with low body temperature. These findings, combined, led the researchers to conclude that neither low metabolic rate nor reduced caloric intake alone are sufficient to slow down blood epigenetic aging. Instead, a drop in body temperature is necessary for the anti-aging effects of torpor.
Although the exact mechanisms linking low body temperature and epigenetic aging are unclear, the team hypothesizes that it may involve the cell cycle, which regulates how cells grow and divide: lower body temperatures can potentially slow down cellular processes, including DNA replication and mitosis. This, over time, may impact cell turnover and aging. With further research, the Hrvatin Lab aims to explore this link in greater depth and shed light on the lingering mystery.