Research Area: Microbiology
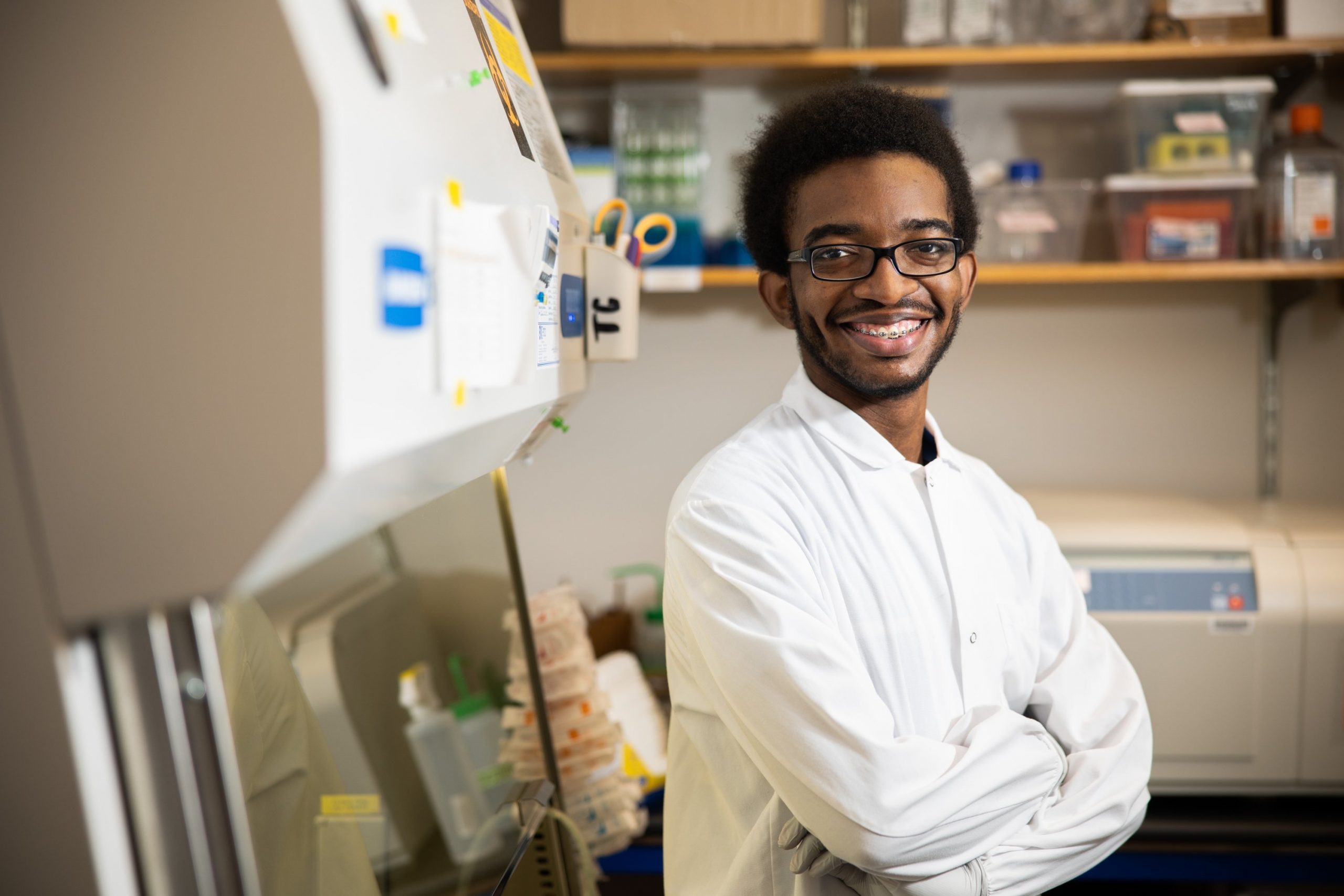
Senior Desmond Edwards has an insatiable curiosity about how the human body works — and how diseases stop it from working.
Leah Campbell | School of Science
November 21, 2021
Desmond Edwards was a little kid when first learned about typhoid fever. Fortunately, he didn’t have the disease. He was looking at a cartoon public health announcement. The cartoon, produced by the Pan American Health Organization, was designed to educate people in his home country of Jamaica about the importance of immunizations for diseases like typhoid. The typhoid character in the cartoon was so unpleasant it gave him nightmares.
Edwards did have his fair share of hospital visits throughout his childhood. But, his own struggles with infection and illness, and those typhoid cartoon nightmares, became his inspiration for pursuing a career studying human disease. At age 6, Edwards was running impromptu baking soda experiments in repurposed glitter containers in his kitchen. Today, he is a senior at MIT, majoring in biology and biological engineering, thanks to a team of dedicated mentors and an insatiable curiosity about how the human body works — or, more accurately, how diseases stop it from working.
Finding a way into research
Edwards knew he wanted to do research but says he assumed that that was something you did after you got your degree. Imagine his surprise, then, upon arriving at MIT in 2018 and meeting classmates who not only had done research, but already had publications. Realizing that he could get a jump-start on his career, he sought out research opportunities and enrolled in the biology class 7.102 (Introduction to Molecular Biology Techniques) for his first-year Independent Activities Period. The class was specifically geared toward first-year students like him with no lab experience.
“It was a great first look at how research is done,” Edwards says of the class. Students took water samples from the Charles River and were expected to identify the strains of bacteria found in those samples using various biological techniques. They looked at the bacteria under a microscope. They examined how the samples metabolized different sources of carbon and determined if they could be stained by different dyes. They even got to try out basic genetic sequencing. “We knew where we were starting. And we knew the end goal,” says Edwards. The in-between was up to them.
Class 7.102 is taught by Mandana Sassanfar, a lecturer in biology and the department’s director of diversity and science outreach. For Sassanfar, the class is also an opportunity to find lab placements for students. In Edwards’ case, she literally led him to the lab of Assistant Professor Becky Lamason, walking up with him one evening to meet a postdoc, Jon McGinn, to talk about the lab and opportunities there. After Edwards expressed his interest to Lamason, she responded within 30 minutes. McGinn even followed up to answer any lingering questions.
“I think that was really what pushed it over the edge,” he says of his decision to take a position in the Lamason lab. “I saw that they were interested not only in having me as someone to help them do research, but also interested in my personal development.”
At the edges of cells and disciplines
The Lamason lab researches the life cycle of two different pathogens, trying to understand how the bacteria move between cells. Edwards has focused on Rickettsia parkeri, a tick-borne pathogen that’s responsible for causing spotted fever. This type of Rickettsia is what biologists call an obligate intracellular pathogen, meaning that it resides within cells and can only survive when it’s in a host. “I like to call it a glorified virus,” Edwards jokes.
Edwards gets excited describing the various ways in which R. parkeri can outsmart its infected host. It’s evolved to escape the phagosome of the cell, the small liquid sac that forms from the cell membrane and engulfs organisms like bacteria that pose a threat. Once it gets past the phagosome and enters the cell, it takes over cellular machinery, just like a virus. At this point of the life cycle, a bacterium will typically replicate so many times that the infected cell will burst, and the pathogen will spread widely. R. parkeri, though, can also spread to uninfected cells directly through the membrane where two cells touch. By not causing a cell to burst, the bacterium can spread without alerting the host to its presence.
“From a disease standpoint, that’s extremely interesting,” says Edwards. “If you’re not leaving the cell or being detected, you don’t see antibodies. You don’t see immune cells. It’s very hard to get that standard immune response.”
In his time in the lab, Edwards has worked on various projects related to Rickettsia, including developing genetic tools to study the pathogen and examining the potential genes that might be important in its life cycle. His projects sit at the intersection of biology and biological engineering.
“For me, I kind of live in between those spaces,” Edwards explains. “I am extremely interested in understanding the mechanisms that underlie all of biology. But I don’t only want to understand those systems. I also want to engineer them and apply them in ways that can be beneficial to society.”
Science for society
Last year, Edwards won the Whitehead Prize from the Department of Biology, recognizing students with “outstanding promise for a career in biological research.” But his extracurricular activities have been driven more by his desire to apply science for tangible social benefits.
“How do you take the science that you’ve done in the lab, in different research contexts, and translate that in a way that the public will actually benefit from it?” he asks.
Science education is particularly important for Edwards, given the educational opportunities he was given to help get to MIT. As a high schooler, Edwards participated in a Caribbean Science Foundation initiative called the Student Programme for Innovation in Science and Engineering. SPISE, as it’s known, is designed to encourage and support Caribbean students interested in careers in STEM fields. The program is modeled on the Minority Introduction to Engineering and Science program (MITES) at MIT. Cardinal Warde, a professor of electrical engineering, is himself from the Caribbean and serves as the faculty director for both MITES and SPISE.
“That experience not only kind of opened my eyes a bit more to what was available, what was in the realm of possibilities, but also provided support to get to MIT,” Edwards says of SPISE. For example, the program helped with college applications and worked with him to secure an internship at a biotech company when he first moved to the United States.
“If education falters, then you don’t replenish the field of science,” Edwards argues. “You don’t get younger generations excited, and the public won’t care.”
Edwards has also taken a leadership role in the MIT Biotechnology Group, a campus-wide student group meant to build connections between the MIT community and thought leaders in industry, business, and academia. For Edwards, the biotech and pharmaceutical industries play a clear role in disease treatment, and he knew he wanted to join the group before he even arrived at MIT. In 2019, he became co-director of the Biotech Group’s Industry Initiative, a program focused on preparing members for industry careers. In 2020, he became undergraduate president, and this year he’s co-president of the entire organization. Edwards speaks proudly of what the Biotech Group has accomplished during his tenure on the executive board, highlighting that they not only have the largest cohort ever this year, but it’s also the first time the group has been majority undergraduate.
Somehow, in between his research and outreach work, Edwards finds time to minor in French, play for the Quidditch team, and serve as co-president on the Course 20 Undergraduate Board, among other activities. It’s a balancing act that Edwards has mastered over his time at MIT because of his genuine excitement and interest in everything that he does.
“I don’t like not understanding things,” he jokes. “That applies to science, but it also extends to people.”
November 3, 2021
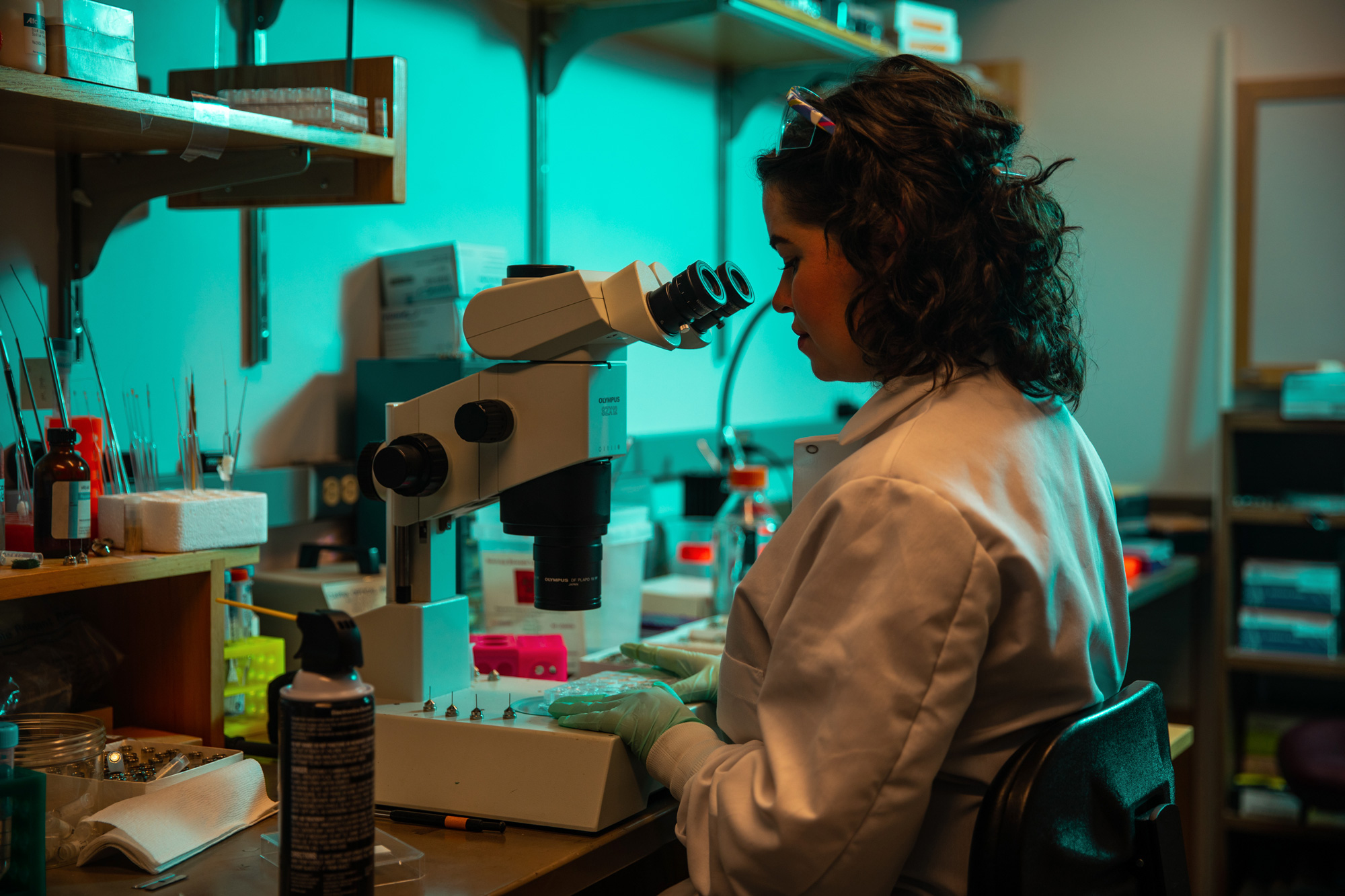
The PhD candidate studies the human microbiome and its proteins, while also championing the Latinx community on MIT’s campus.
Hannah Meiseles | MIT News Office
September 28, 2021
Raised in Tampa, Florida, Lindsey Backman takes pride in her family’s history and its role in the vibrant Cuban American community there. She remembers the weekends she would spend as a kid, getting café con leche with her grandparents and dancing in the studio with her friends. The cultural experiences she shared with friends, family, and neighbors growing up helped her feel comfortable being herself while growing up, and showed her from an early age how valuable a welcoming community could be to a person’s success.
Backman went on to pursue her BS in chemistry at the University of Florida. Surrounded by a diverse community, she felt supported as she leaned deeper into her interest in science. She was soon nominated to a program that matched students from underrepresented backgrounds in STEM with a university professor to pursue a summer research project. Although Backman was still uncertain about going to another university to do lab research, with encouragement from her department she gave the program a chance.
Backman matched with Professor Catherine Drennan at MIT to work on visualizing structural biology and took part in the MIT Summer Research Program in Biology (MSRP-Bio). The research clicked with her immediately and became a turning point; Backman returned to participate in the lab the following summer and then applied to graduate school.
“Getting nominated to the program changed my life. I certainly wouldn’t have applied to MIT otherwise,” says Backman. “At first, I was convinced I wouldn’t fit in, but soon found myself surrounded by people as passionate about science as I was. I knew I was in the right place.”
Uncovering secrets about the human microbiome
Today, Backman is a graduate student in the Drennan lab and researches the chemistry of the human microbiome, a collection of gut microbes essential to sustaining the body. Backman is interested in how certain bacteria can outcompete other strains by producing unique proteins that process abundant nutrients or repair broken enzymes. Her use of X-ray crystallography has helped her produce atomic models that shed light on the structure of these proteins.
One type of protein Backman and her team have characterized is called a spare part protein. When produced, this protein can help restore a broken enzyme’s ability to catalyze essential reactions. “When fixing a car with a flat tire, you would replace the tire and not the whole car. A similar strategy is being used here. These spare-part proteins act to bind and restore the activity of the enzyme completely,” she says.
Over the years, Backman has seen the depth of questions surrounding the microbiome grow. Scientists have begun to recognize how important the microbiome is to human health. “Ever since my first summer research experience at MIT, I’ve been dedicated to studying this one unique repair mechanism,” says Backman. “We’ve gone from solving the structure of the proteins to now understanding how the mechanism works. But there’s still so much more to learn — we have started to suspect these repair mechanisms speak to a broader motif in other enzymes as well.”
Backman and her team have also been leaders in characterizing how an important enzyme, called hydroxy-L-proline dehydratase (HypD), performs its unusual chemistry. This abundant enzyme takes hydroxyproline, a common nutrient in the gut, and can obtain a competitive advantage by using it as a nutrient and source of energy.
“Only a unique subset of bacteria can process hydroxyproline. On the clinical side, we have seen during infection that virulent bacteria with this ability, such as C. difficile, will start rapidly consuming hydroxyproline to proliferate,” says Backman. “Conversely, we could one day create antibiotics that specifically inhibit HypD without killing our beneficial bacteria.”
Encouraging the future of science
Outside of her research, Backman cares deeply about serving and being a part of the Latinx community on campus. She helped co-found the MIT Latinx Graduate Student Association and has served for four years as a graduate resident assistant for La Casa, the Latinx undergraduate living community at New House. “La Casa is a really tight-knit and familial community,” says Backman. “Some of our original freshmen are now seniors, so it’s been really rewarding to see their whole transition throughout college. I love getting to watch students explore and come to realize what they’re passionate about.”
Backman has also been instrumental in spurring equity initiatives on campus. She is currently a student representative for the MIT Department of Chemistry Diversity, Equity, and Inclusion Committee and has worked to implement programs that support the success of underrepresented groups on campus. Her five years of service as an MIT Chemistry Access Program mentor have encouraged many underrepresented undergraduate students to pursue chemistry graduate programs. For all her hard work at improving MIT’s campus, Backman recently received the Hugh Hampton Young Fellowship.
In the future, Backman aspires to continue researching the microbiome and mentoring students by becoming a professor. She hopes to continue the cycle and inspire more young scientists to recognize their inner potential. “I was never one of those kids that knew I wanted to be a scientist someday. My PI completely changed my life, and I would not be at MIT today without her,” she says. “Having mentors that believe in you at critical points in your life can make all the difference.”
“I think there’s this wrong assumption that diversity initiative work takes away from time that could be spent doing science. In my mind, we need to recognize how these things go hand in hand,” says Backman.
“The only way we’re going to get the best scientists is by creating a healthier, more diverse environment where people of all backgrounds feel welcomed. It’s only when people feel comfortable that they can make their greatest contributions to the field.”
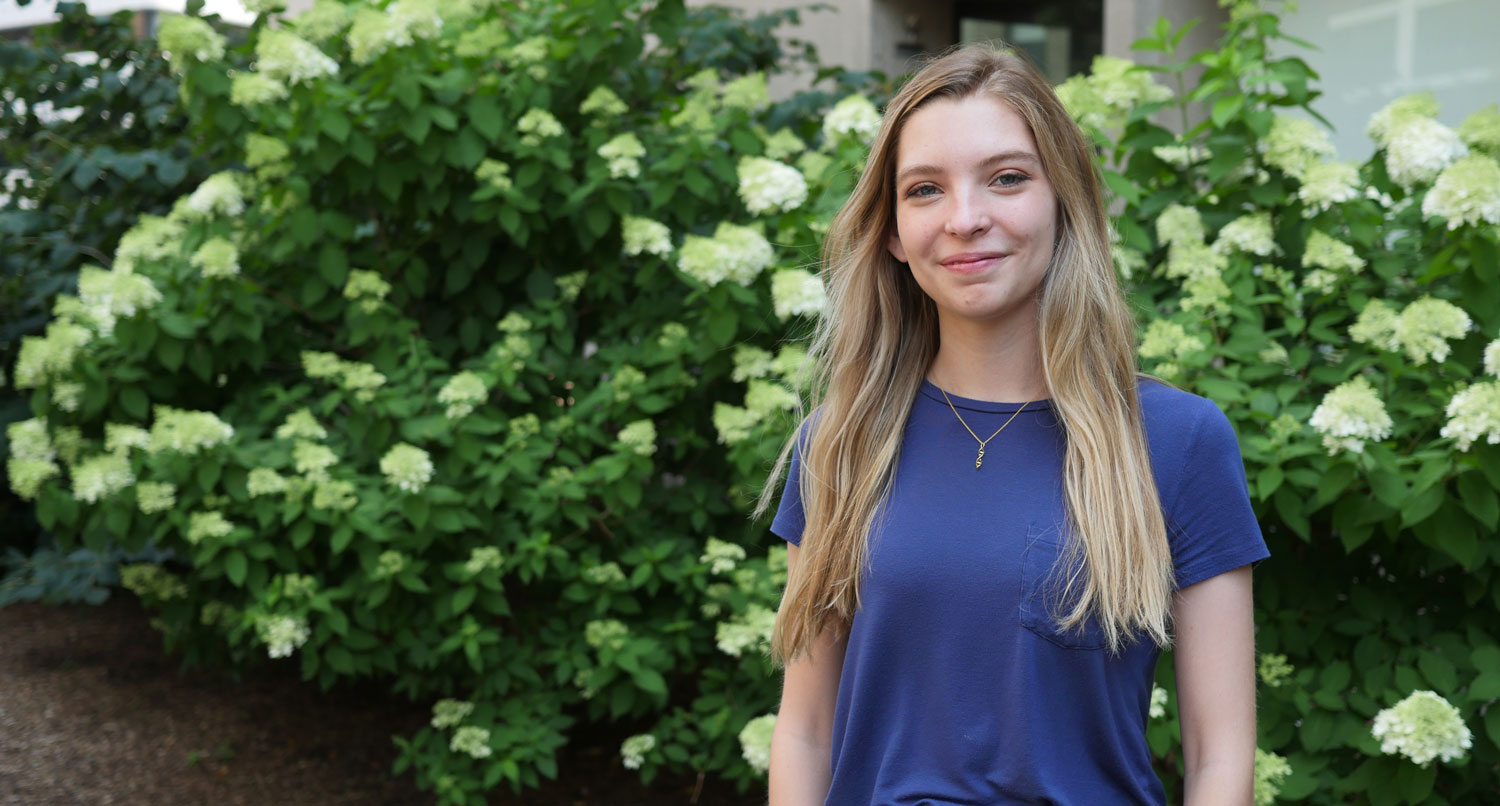
Bailey Bowcutt investigated COVID-19 cases in rural Wyoming before coming to MIT for the summer and applying her knowledge to a new cellular invader.
Raleigh McElvery
July 23, 2021
The first time Bailey Bowcutt saw a lab it was nothing like she expected. Rather than a stark, sterile setting with sullen figures floating around like ghosts in white lab coats, the atmosphere was cordial and the dress casual. Some scientists even sported vibrant shirts with Marvel characters. A high school senior on a class field trip, Bowcutt couldn’t have predicted that the next time she’d set foot in the Wyoming Public Health Laboratory she’d no longer be a visitor, but a researcher performing diagnostic testing during a global pandemic. Now, as COVID-19 restrictions begin to lift, she’s taking the research tools she’s learned to Cambridge, Massachusetts to complete the Bernard S. and Sophie G. Gould MIT Summer Research Program in Biology (BSG-MSRP-Bio) and investigate how other types of pathogens spread.
Growing up in rural Wyoming, Bowcutt had little exposure to science because there were few research institutes close by. But watching family members suffer from gastrointestinal illness and other infections spurred her to pursue a degree in microbiology at Michigan State University (MSU). Shortly after she arrived on campus in the fall of 2019, she joined Shannon Manning’s lab studying antibiotic resistance in cattle.
Cows are prone to contracting a bacterial infection of the udder called mastitis. (In humans, a similar inflammation can occur in breast tissue.) Manning’s lab is looking at how antibiotic treatments affect the bovine gut microbiome and emergence of antibiotic resistance genes. Bowcutt’s role was to help identify these super bugs inside the cows’ gastrointestinal tracts.
“I got to go to the farm to take samples, which involved a glove that goes all the way up to the shoulder and some invasive maneuvers inside cows,” she explains. “Luckily, I was just the bag holder!”
Intimate sample collection aside, Bowcutt was excited about the work because it combined agriculture and human health research to solve issues plaguing rural communities. But her time on the farm was cut short when COVID-19 cases climbed in early 2020. She headed back to her home in Wyoming to begin remote MSU classes and, reminiscing about her field trip to the Wyoming Public Health Laboratory, reached out to the director to see if there were any internship opportunities.
“I’d barely learned how to do science at that point, but they needed people who could handle a pipette, so they took me,” she says. “I ended up being one of the first people there helping with COVID research, and I stayed for about a year-and-a-half while I took online classes.”
The lab would receive nasopharyngeal swabs from COVID-19 patients, and Bowcutt’s first task was to help extract RNA from the samples. Later, she transitioned to another project, which required performing PCR on untreated wastewater samples to glean a population-level understanding of where COVID-19 outbreaks were occurring.
She began toying with the idea of pursuing a PhD, but wasn’t sure what it would entail. So, in early 2021, she started Googling summer science programs and stumbled on BSG-MSRP-Bio. She was accepted, and paired with one of the very labs that had caught her eye online: assistant professor Becky Lamason’s group.
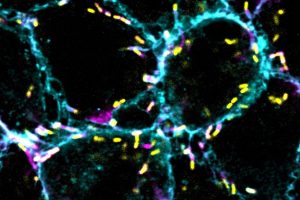
“If you’ve ever seen microscopy pictures from the Lamason lab, they’re just so beautiful,” Bowcutt explains. Beautiful, yes — but she would soon learn these snapshots capture a chilling cellular invasion and molecular heist.
The Lamason lab watches malicious bacteria as they hijack molecules in human host cells to build long tails, rocket around, and punch through the cell membrane to spread. Bowcutt’s mentor, graduate student Yamilex Acevedo-Sánchez, focuses on the food-borne bacterium Listeria monocytogenes, which targets the gastrointestinal tract. Acevedo-Sánchez’s research aims to understand the host cell pathways that Listeria commandeers to move from one cell to the next in a process called cell-to-cell spread.
Together, Acevedo-Sánchez and Bowcutt are investigating several proteins in the human host cell involved in cellular transport and membrane remodeling (Caveolin-1, Pacsin2, and Fes), which could regulate Listeria’s spread. Over the summer, the duo has been adjusting the levels of these proteins and observing what happens to Listeria’s ability to move from cell-to-cell.
Bowcutt spends most of her days doing Western blots; growing Listeria and mammalian cells; and combining immunofluorescence assays with fixed and live cell microscopy to take her own striking microscopy images and movies of the parasites.
“I expected the work environment at MIT to be very intense, but everyone has been really friendly and willing to answer questions,” she says. “Some of my favorite experiences have just been in the lab while everyone is bustling around. It’s a welcome change after so much COVID-19 isolation.”
Now that the COVID-era occupancy restrictions have lifted, Bowcutt’s lab bench neighbor is Lamason herself. “She’s next to me doing experiments all the time,” Bowcutt explains, “which is cool because she’s really engaging with the research in the same way we are.”
Bowcutt says her summer experience has given her some much-needed practice designing research questions and devising the experiments to answer them. She’s also acquired a new skill she didn’t anticipate: interpreting ambiguous results and developing follow-up experiments to clarify them.
These days, the prospect of a PhD seems much less intimidating. In fact, the Lamason lab has done more than simply pique Bowcutt’s interested in fundamental biology research. She’s now considering ways to combine her microbiology skills with her interest in rural health care.
“I didn’t expect to see this much growth in myself,” she says, “and I know it’s making me a better scientist. I’m excited to return to MSU in the fall because I feel like I can do so much more now — and I would totally do it again.”
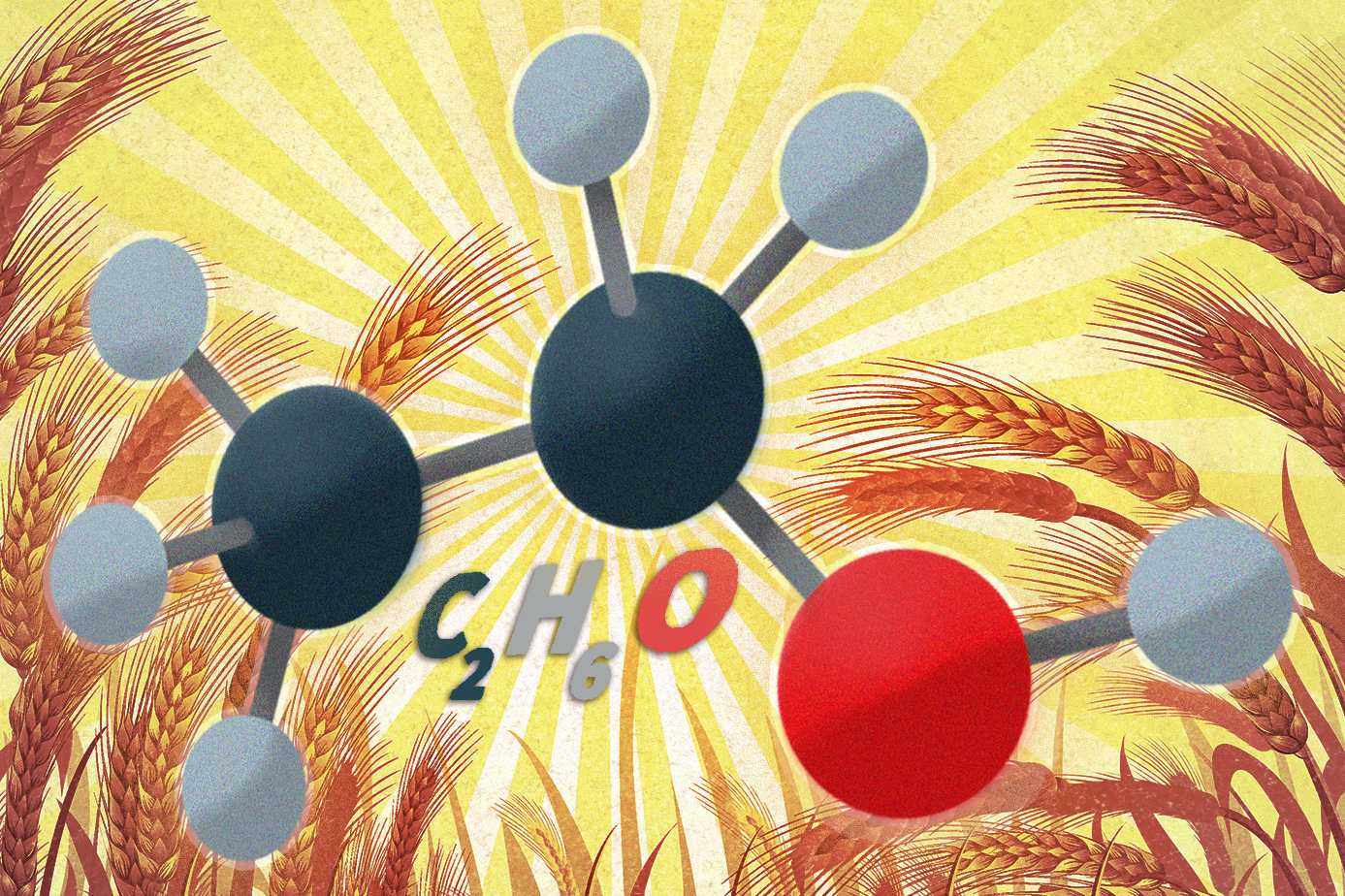
By making the microbes more tolerant to toxic byproducts, researchers show they can use a wider range of feedstocks, beyond corn.
Anne Trafton | MIT News Office
June 28, 2021
Boosting production of biofuels such as ethanol could be an important step toward reducing global consumption of fossil fuels. However, ethanol production is limited in large part by its reliance on corn, which isn’t grown in large enough quantities to make up a significant portion of U.S. fuel needs.
To try to expand biofuels’ potential impact, a team of MIT engineers has now found a way to expand the use of a wider range of nonfood feedstocks to produce such fuels. At the moment, feedstocks such as straw and woody plants are difficult to use for biofuel production because they first need to be broken down to fermentable sugars, a process that releases numerous byproducts that are toxic to yeast, the microbes most commonly used to produce biofuels.
The MIT researchers developed a way to circumvent that toxicity, making it feasible to use those sources, which are much more plentiful, to produce biofuels. They also showed that this tolerance can be engineered into strains of yeast used to manufacture other chemicals, potentially making it possible to use “cellulosic” woody plant material as a source to make biodiesel or bioplastics.
“What we really want to do is open cellulose feedstocks to almost any product and take advantage of the sheer abundance that cellulose offers,” says Felix Lam, an MIT research associate and the lead author of the new study.
Gregory Stephanopoulos, the Willard Henry Dow Professor in Chemical Engineering, and Gerald Fink, the Margaret and Herman Sokol Professor at the Whitehead Institute of Biomedical Research and the American Cancer Society Professor of Genetics in MIT’s Department of Biology, are the senior authors of the paper, which appears today in Science Advances.
Boosting tolerance
Currently, around 40 percent of the U.S. corn harvest goes into ethanol. Corn is primarily a food crop that requires a great deal of water and fertilizer, so plant material known as cellulosic biomass is considered an attractive, noncompeting source for renewable fuels and chemicals. This biomass, which includes many types of straw, and parts of the corn plant that typically go unused, could amount to more than 1 billion tons of material per year, according to a U.S. Department of Energy study — enough to substitute for 30 to 50 percent of the petroleum used for transportation.
However, two major obstacles to using cellulosic biomass are that cellulose first needs to be liberated from the woody lignin, and the cellulose then needs to be further broken down into simple sugars that yeast can use. The particularly aggressive preprocessing needed generates compounds called aldehydes, which are very reactive and can kill yeast cells.
To overcome this, the MIT team built on a technique they had developed several years ago to improve yeast cells’ tolerance to a wide range of alcohols, which are also toxic to yeast in large quantities. In that study, they showed that spiking the bioreactor with specific compounds that strengthen the membrane of the yeast helped yeast to survive much longer in high concentrations of ethanol. Using this approach, they were able to improve the traditional fuel ethanol yield of a high-performing strain of yeast by about 80 percent.
In their new study, the researchers engineered yeast so that they could convert the cellulosic byproduct aldehydes into alcohols, allowing them to take advantage of the alcohol tolerance strategy they had already developed. They tested several naturally occurring enzymes that perform this reaction, from several species of yeast, and identified one that worked the best. Then, they used directed evolution to further improve it.
“This enzyme converts aldehydes into alcohols, and we have shown that yeast can be made a lot more tolerant of alcohols as a class than it is of aldehydes, using the other methods we have developed,” Stephanopoulos says.
Yeast are generally not very efficient at producing ethanol from toxic cellulosic feedstocks; however, when the researchers expressed this top-performing enzyme and spiked the reactor with the membrane-strengthening additives, the strain more than tripled its cellulosic ethanol production, to levels matching traditional corn ethanol.
Abundant feedstocks
The researchers demonstrated that they could achieve high yields of ethanol with five different types of cellulosic feedstocks, including switchgrass, wheat straw, and corn stover (the leaves, stalks, and husks left behind after the corn is harvested).
“With our engineered strain, you can essentially get maximum cellulosic fermentation from all these feedstocks that are usually very toxic,” Lam says. “The great thing about this is it doesn’t matter if maybe one season your corn residues aren’t that great. You can switch to energy straws, or if you don’t have high availability of straws, you can switch to some sort of pulpy, woody residue.”
The researchers also engineered their aldehyde-to-ethanol enzyme into a strain of yeast that has been engineered to produce lactic acid, a precursor to bioplastics. As it did with ethanol, this strain was able to produce the same yield of lactic acid from cellulosic materials as it does from corn.
This demonstration suggests that it could be feasible to engineer aldehyde tolerance into strains of yeast that generate other products such as diesel. Biodiesels could potentially have a big impact on industries such as heavy trucking, shipping, or aviation, which lack an emission-free alternative like electrification and require huge amounts of fossil fuel.
“Now we have a tolerance module that you can bolt on to almost any sort of production pathway,” Stephanopoulos says. “Our goal is to extend this technology to other organisms that are better suited for the production of these heavy fuels, like oils, diesel, and jet fuel.”
The research was funded by the U.S. Department of Energy and the National Institutes of Health.
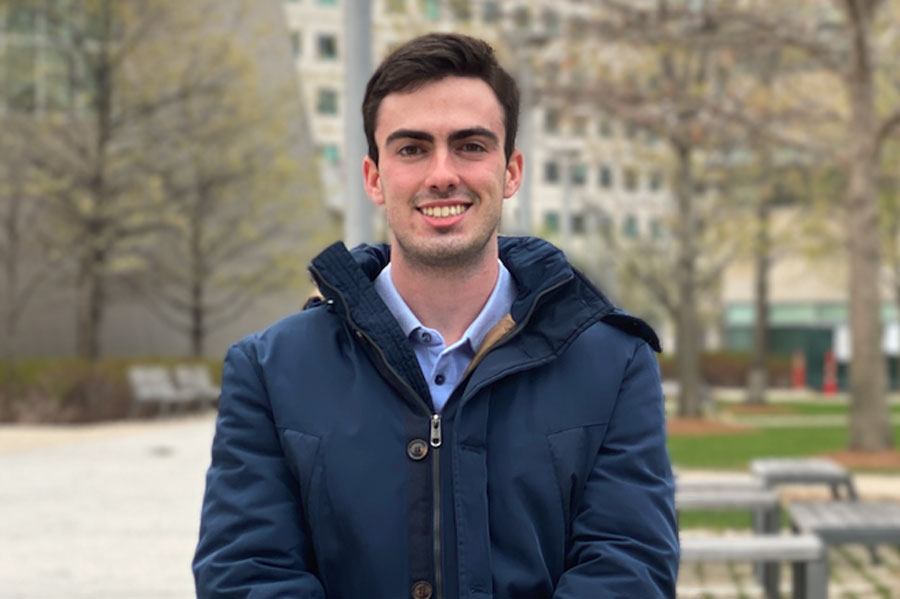
MIT Biology junior Eduardo Canto tinkered with science long before he started studying Treacher Collins syndrome in the Calo lab.
Saima Sidik | Department of Biology
May 19, 2021
In seventh grade, Eduardo Canto wanted a dog. His mom said no, though. She didn’t want to spend her days vacuuming fur. They reached a compromise: Canto was allowed to have pet fish. Soon Canto’s disappointment with his new pets turned to curiosity. While he couldn’t train the fish to sit or roll over, he decided that breeding the fish could be a fun pastime.
An internet search told Canto that some aquarists use dried Indian almond leaves, a traditional Asian herbal remedy, to stimulate fish breeding, although no one is quite sure how the leaves do this. However, finding Indian almond leaves presented a problem for a kid without an Amazon account living far from the tree’s native habitat. On a whim, Canto picked up some similar-looking leaves in a park near his house in Puerto Rico. He knew they weren’t from an Indian almond tree, but he put them in the tank anyhow, just to see what would happen. A few days later, he noticed a collection of eggs attached to the bottom of a leaf!
Canto often took on little experiments like this, which caused his grandfather to predict early on that he would have a scientific career. Eight years after the breeding endeavor, Canto is fulfilling his grandfather’s prediction by studying Course 7 (Biology) at MIT, where he’s currently in his third year of a bachelor’s degree. Once again, fish have come into Canto’s life — he’s working in Eliezer Calo’s lab, where researchers use zebrafish to study a genetic disorder called Treacher Collins syndrome, which causes deformities in eyes, ears, cheekbones, and chins.
Throughout middle school and high school, Canto dipped his toes into many scientific disciplines. School science fairs motivated him to build a dry ice-powered trolley, a solar-powered water heater, and start a vegetable garden.
Sometimes, he admits, his motivation for joining science clubs wasn’t lofty. “I joined the math club because I got to miss a day of school every year for their annual competition,” he says with a laugh. But he also talks excitedly about his early experiments, particularly in biology. “I’ve always loved working with my hands,” he says.
Canto’s father, a medical doctor, encouraged his son’s interest by letting Canto shadow him at work. He also started a molecular biology summer program at Canto’s high school that taught students how to pipette and do simple experiments. By the time Canto applied to college, he was convinced he wanted to study biology, and MIT drew his attention because of its reputation as a top science school with excellent biology teachers. He knew it was the right choice for him when he attended Campus Preview Weekend, and found a large Puerto Rican community ready to welcome him. Even far from the island, he felt at home.
Canto has kept up with his roots since joining MIT by playing on a soccer team for Puerto Rican students. He’s also become part of a new community in a lab run by Eliezer Calo — who is a Puerto Rican himself. The lab is interested in ribosomes, the molecular machines that build proteins. Treacher Collins syndrome arises when cells can’t make ribosomes properly, and Canto wants to understand why that is.
Before Canto joined the Calo lab, the group had already started studying a protein called DDX21 that’s involved in making ribosomes in both humans and zebrafish. When genetic mutations in zebrafish cause DDX21 to go to the wrong part of the cell, the fish develop jaw deformations that mirror Treacher Collins syndrome. The Calo lab thinks cells with mislocalized DDX21 probably don’t produce ribosomes as well as normal cells, but they’re still testing this hypothesis.
Transcript
Canto wants to probe the relationship between DDX21 and Treacher Collins syndrome further, but fish reproduce slowly, so they’re not ideal organisms for his research. Instead, he’s built a strain of Escherichia coli bacteria that carry DDX21 in place of the equivalent bacterial gene. DDX21 helps these bacteria survive the stress associated with cold temperatures, so without it, the bacteria will die in the cold. Canto hopes to take advantage of this trait by finding small molecules that stop the bacteria from growing at low temperatures — just like a DDX21 mutation would. Studying how these molecules bind DDX21 will help him understand which parts of this protein are important for its function.
The possibility that this work will one day reveal how Treacher Collins syndrome develops in patients is rewarding to Canto, and in fact he hopes helping patients will soon become his life’s focus. He wants to attend medical school, and eventually become a doctor. The human physiology class he took last semester was one of his favorites, even though it was over Zoom due to the COVID-19 pandemic. Becoming a doctor will let him help others while studying topics he finds fascinating. “Medicine is like biology on steroids!” he says.
And who knows — one day after he’s a doctor, maybe he’ll even get that pet he’s always wanted. But unlike Canto’s interest in biology, some of his interests have evolved over time. These days, he prefers cats over dogs.
Photo credit: Saima Sidik
Posted: 5.19.21
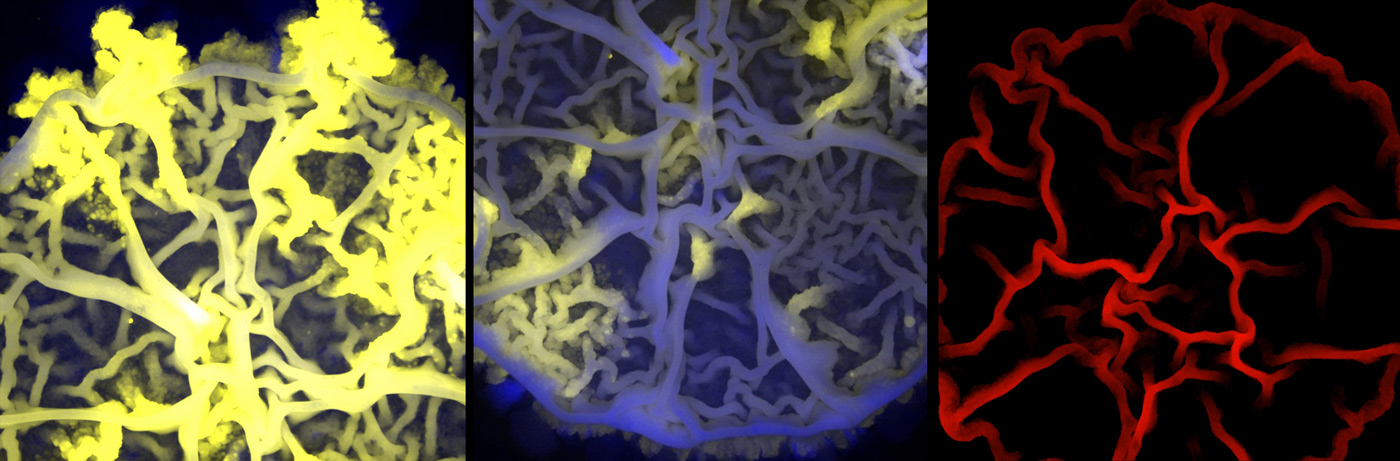
Raleigh McElvery
March 12, 2021
Scientists have a term for genes that spread themselves throughout a population at any cost: “selfish” DNA. One way that these genes transmit through bacterial communities is via a type of bacterial sex called conjugation. When one bacterium makes contact with another, DNA from the host cell can be injected into a recipient cell.
Alan Grossman’s lab at the MIT Department of Biology studies a small but selfish chunk of DNA called ICEBs1. His group has identified several ways in which this so-called mobile genetic element actually benefits its host bacterium as it fights to spread. Building off this body of work, Grossman’s lab collaborated with colleagues at Tel Aviv University on a new study recently published in eLife. The international team found that ICEBs1 contains one gene in particular, which allows the host cell to continue dividing in densely-packed microbial communities. This helps the host to grow in conditions where nutrients are scarce, while also potentially helping ICEBs1 to propagate.
“Mobile genetic elements like ICEBs1 are found in the chromosomes of many different types of bacteria,” says Grossman, department head and co-senior author on the study. “Studying these elements — how they spread and how they affect their host cells — is critical for understanding the evolution of bacteria, engineering some types of bacteria to do useful things, and possibly preventing the deleterious effects caused by harmful bacteria.”
Like many DNA segments on the move, ICEBs1 includes genes that encode the molecular machinery required to transfer itself from one cell to the next. But mobile genetic elements can also contain “cargo” genes that bestow the host bacterium with new traits, such as antibiotic resistance. However, in many cases, the properties a cargo gene will endow are hard to predict.
“The host cell can get a lot of new genes in a hurry through mobile genetic elements like ICEBs1, and there’s a lot we still don’t know about the types of phenotypes cargo genes confer,” says the study’s first author, Joshua Jones PhD ’20. “The array of possible traits is probably a lot more diverse than we currently appreciate.”
To investigate the changes that ICEBs1 triggers in the host cell, Jones and colleagues examined large microbial communities called biofilms. These form when many bacteria aggregate on a surface and secrete a slimy “glue” made of sugar, proteins, and DNA that encases the population. Common examples of biofilms include dental plaque, the sludge that coats the inside of pipes, or the deleterious infections that form on surgical implants in patients’ bodies.
Because there are so many bacteria in close contact, biofilms are hot spots for exchanging mobile genetic elements like ICEBs1. However, secreting the materials needed to produce the slimy glue can rapidly deplete resources. As a result, bacteria in a biofilm do not always have the capacity to grow, divide, and potentially spread ICEBs1. Instead, certain types of rod-shaped bacteria begin to produce spores that are analogous to plant seeds. This process, called sporulation, enables these bacteria to become dormant and survive extreme conditions.
Jones found that Bacillus subtilis bacteria containing ICEBs1 were delayed in contributing to the biofilm glue, and also delayed in producing dormant spores. As a result, these bacteria could continue dividing for longer than bacteria without ICEBs1 — increasing the number of bacteria with ICEBs1 and the likelihood that ICEBs1 would spread. The researchers were able to pinpoint one ICEBs1 cargo gene in particular, called Development Inhibitor (devI), that triggered this delay in both biofilm development and sporulation.
“In a way, the cells with ICEBs1 are ‘cheating’ by delaying sporulation and not contributing to the greater good of the biofilm community,” Jones says. But, he explains, they can get away with it because the devI pathway only initiates when ICEBs1-containing cells are the minority in a microbial population. In order to spread as widely as possible, it’s best for ICEBs1 to transfer to new cells that don’t already contain existing copies. Furthermore, accumulating duplicate copies can have detrimental effects on ICEBs1 itself.
“It’s a very clever system for assessing the situation around the cell, and deciding whether it’s worthwhile for ICEBs1 to attempt to transfer,” Jones adds.
Next, the Grossman lab plans to determine precisely how devI exerts its effects on biofilm formation and sporulation. They suspect that other ICEBs1-like elements may also use genes analogous to devI to execute similar propagation strategies. Probing such “cheating” tactics orchestrated by selfish genes will help scientists better understand microbial evolution and, eventually, perhaps even inspire drugs to disrupt harmful biofilms, like those that form around surgical implants.
February 6, 2021
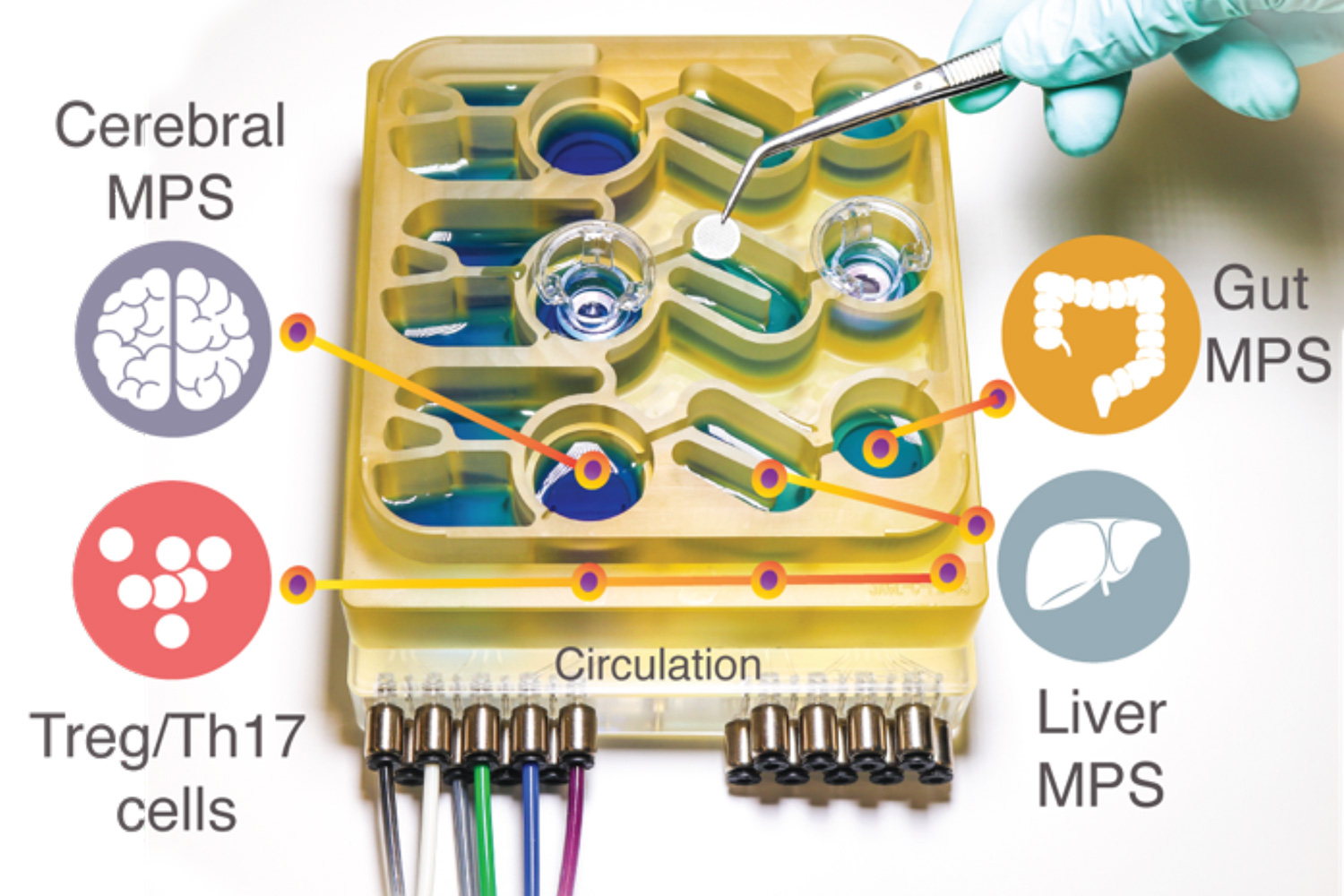
“Organs-on-a-chip” system sheds light on how bacteria in the human digestive tract may influence neurological diseases.
Anne Trafton | MIT News Office
January 29, 2021
In many ways, our brain and our digestive tract are deeply connected. Feeling nervous may lead to physical pain in the stomach, while hunger signals from the gut make us feel irritable. Recent studies have even suggested that the bacteria living in our gut can influence some neurological diseases.
Modeling these complex interactions in animals such as mice is difficult to do, because their physiology is very different from humans’. To help researchers better understa nd the gut-brain axis, MIT researchers have developed an “organs-on-a-chip” system that replicates interactions between the brain, liver, and colon.
Using that system, the researchers were able to model the influence that microbes living in the gut have on both healthy brain tissue and tissue samples derived from patients with Parkinson’s disease. They found that short-chain fatty acids, which are produced by microbes in the gut and are transported to the brain, can have very different effects on healthy and diseased brain cells.
“While short-chain fatty acids are largely beneficial to human health, we observed that under certain conditions they can further exacerbate certain brain pathologies, such as protein misfolding and neuronal death, related to Parkinson’s disease,” says Martin Trapecar, an MIT postdoc and the lead author of the study.
Linda Griffith, the School of Engineering Professor of Teaching Innovation and a professor of biological engineering and mechanical engineering, and Rudolf Jaenisch, an MIT professor of biology and a member of MIT’s Whitehead Institute for Medical Research, are the senior authors of the paper, which appears today in Science Advances.
The gut-brain connection
For several years, Griffith’s lab has been developing microphysiological systems — small devices that can be used to grow engineered tissue models of different organs, connected by microfluidic channels. In some cases, these models can offer more accurate information on human disease than animal models can, Griffith says.
In a paper published last year, Griffith and Trapecar used a microphysiological system to model interactions between the liver and the colon. In that study, they found that short-chain fatty acids (SCFAs), molecules produced by microbes in the gut, can worsen autoimmune inflammation associated with ulcerative colitis under certain conditions. SCFAs, which include butyrate, propionate, and acetate, can also have beneficial effects on tissues, including increased immune tolerance, and they account for about 10 percent of the energy that we get from food.
In the new study, the MIT team decided to add the brain and circulating immune cells to their multiorgan system. The brain has many interactions with the digestive tract, which can occur via the enteric nervous system or through the circulation of immune cells, nutrients, and hormones between organs.
Several years ago, Sarkis Mazmanian, a professor of microbiology at Caltech, discovered a connection between SCFAs and Parkinson’s disease in mice. He showed that SCFAs, which are produced by bacteria as they consume undigested fiber in the gut, sped up the progression of the disease, while mice raised in a germ-free environment were slower to develop the disease.
Griffith and Trapecar decided to further explore Mazmanian’s findings, using their microphysiological model. To do that, they teamed up with Jaenisch’s lab at the Whitehead Institute. Jaenisch had previously developed a way to transform fibroblast cells from Parkinson’s patients into pluripotent stem cells, which can then be induced to differentiate into different types of brain cells — neurons, astrocytes, and microglia.
More than 80 percent of Parkinson’s cases cannot be linked to a specific gene mutation, but the rest do have a genetic cause. The cells that the MIT researchers used for their Parkinson’s model carry a mutation that causes accumulation of a protein called alpha synuclein, which damages neurons and causes inflammation in brain cells. Jaenisch’s lab has also generated brain cells that have this mutation corrected but are otherwise genetically identical and from the same patient as the diseased cells.
Griffith and Trapecar first studied these two sets of brain cells in microphysiological systems that were not connected to any other tissues, and found that the Parkinson’s cells showed more inflammation than the healthy, corrected cells. The Parkinson’s cells also had impairments in their ability to metabolize lipids and cholesterol.
Opposite effects
The researchers then connected the brain cells to tissue models of the colon and liver, using channels that allow immune cells and nutrients, including SCFAs, to flow between them. They found that for healthy brain cells, being exposed to SCFAs is beneficial, and helps them to mature. However, when brain cells derived from Parkinson’s patients were exposed to SCFAs, the beneficial effects disappeared. Instead, the cells experienced higher levels of protein misfolding and cell death.
These effects were seen even when immune cells were removed from the system, leading the researchers to hypothesize that the effects are mediated by changes to lipid metabolism.
“It seems that short-chain fatty acids can be linked to neurodegenerative diseases by affecting lipid metabolism rather than directly affecting a certain immune cell population,” Trapecar says. “Now the goal for us is to try to understand this.”
The researchers also plan to model other types of neurological diseases that may be influenced by the gut microbiome. The findings offer support for the idea that human tissue models could yield information that animal models cannot, Griffith says. She is now working on a new version of the model that will include micro blood vessels connecting different tissue types, allowing researchers to study how blood flow between tissues influences them.
“We should be really pushing development of these, because it is important to start bringing more human features into our models,” Griffith says. “We have been able to start getting insights into the human condition that are hard to get from mice.”
The research was funded by DARPA, the National Institutes of Health, the National Institute of Biomedical Imaging and Bioengineering, the National Institute of Environmental Health Sciences, the Koch Institute Support (core) Grant from the National Cancer Institute, and the Army Research Office Institute for Collaborative Biotechnologies.