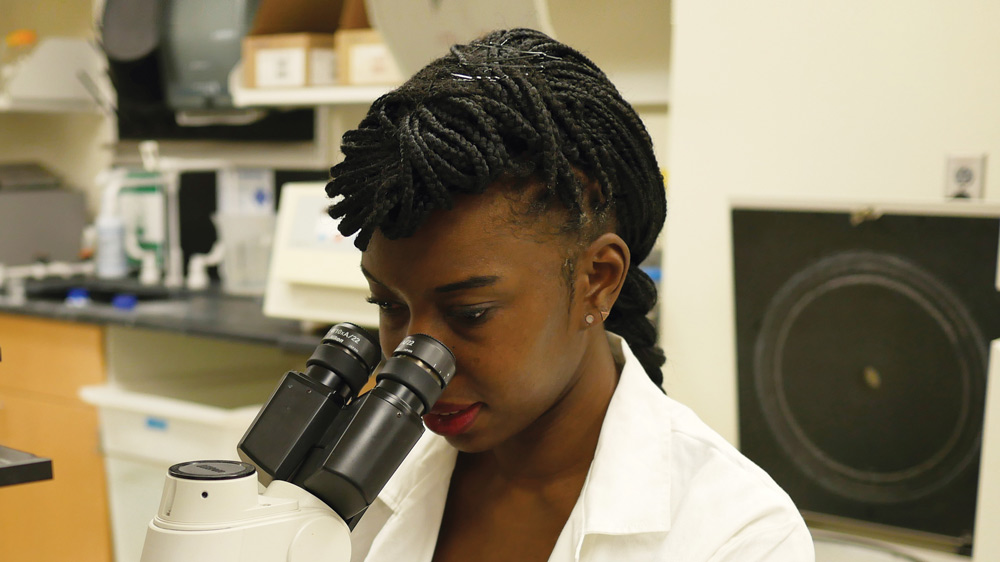
Graduate student Faye-Marie Vassel investigates a protein that helps cells tolerate DNA damage, sharing her expertise with budding scientists to further STEM education
Raleigh McElvery
December 8, 2017
Combatting chemotherapy resistance
Graduate student Faye-Marie Vassel investigates a protein that helps cells tolerate DNA damage, sharing her expertise with budding scientists to further STEM education
Raleigh McElvery
Faye-Marie Vassel has a protein. Well, as a living entity, technically she has many, but just one she affectionately refers to as her own. “My protein, REV7.” And it makes sense — if you were hard at work characterizing a single protein for all six years of your graduate career, you’d be pretty attached, too. Plus, the stakes are high. REV7, which aids in DNA damage repair, could ultimately provide insight into ways to combat chemotherapy resistance.
Although Vassel’s mother trained as an OB/GYN in Russia before moving to the U.S., serving as what Vassel describes as a “quiet” scientific role model, Vassel spent her early childhood emulating her father, a social worker, and engrossed in the social sciences. She intended to one day work in science policy — until high school when she joined an after-school program at the American Museum of Natural History in New York City, and discovered an additional interest.
Here, Vassel took a series of molecular biology classes and met her first female research mentor, a postdoctoral fellow at Rockefeller University, who encouraged her to participate in another, more advanced science program funded by the National Science Foundation.
“I initially had my doubts, but just having that support changed everything,” Vassel says. “That was my first time doing research of any kind, and I got a sense of the sheer diversity of potential research projects. That’s also when I heard there was something called biophysics.”
From that point on, Vassel was hooked. As an undergraduate at Stony Brook University, she initially declared a major in physics before switching to biochemistry. Later, when it came time to select a graduate school, she was split between MIT and the University of California, Berkeley. As she recalls, MIT’s graduate preview weekend made all the difference.
“I had the chance to stay with biology students and speak with professors,” she says. “The whole experience made the department seem personal, and demystified the graduate school process by making it more tangible.”
She proposed a joint position between two labs: Graham Walker’s lab, based in Building 68, and Michael Hemann’s lab situated in the Koch Institute for Integrative Cancer Research. Walker’s lab focuses on microbiology, DNA repair, and antibiotic resistance, while Hemann’s lab investigates chemotherapy resistance in hopes of improving cancer therapies. After stumbling upon one of their joint papers, Vassel decided she’d like to combine the two.
“It’s invaluable to have both perspectives,” she says. “Mike’s lab just celebrated its 10th anniversary, while Graham‘s just had its 35th. It’s been interesting seeing the different ways they approach their respective research questions, because they were trained in such different scientific eras.”
Although Vassel is currently the only student formally working in both labs, the collaboration between Walker and Hemann, aimed at combatting chemotherapy resistance, has been ongoing.
Frontline chemotherapies, including one anticancer agent called cisplatin, kill cancer cells by damaging their DNA and preventing them from synthesizing new genetic material. Just how sensitive cancer cells are to cisplatin — and therefore how effective the treatment is — depends on whether the cell can repair the damage and bypass DNA-damage induced cell death. In some cases, cells increase production of “translesion polymerases,” which are specialized DNA polymerases that can help cells tolerate certain kinds of DNA damage by synthesizing across from damaged DNA or DNA bound to a carcinogen.
Vassel’s protein, REV7, is a structural subunit of one key translesion polymerase, and its expression is deregulated in many different cancer cells. As Vassel suggests, if one aspect of these translesion polymerases — say, the REV7 subunit — could be altered to hinder repair, then perhaps cancer-ridden cells could regain drug sensitivity.
Thanks to recently-developed CRISPR-Cas9 gene editing techniques, Vassel has removed REV7 entirely from drug resistant lung cancer cells, and watched as cisplatin sensitivity was restored. She also conducted rescue experiments, adding REV7 back into cell lines lacking the protein to see whether those cells become resistant to the drug once again. Most recently, she has been working in murine models to see whether REV7 has similar effects in a living system.
If her hypothesis is correct, REV7 would be a powerful target for drug development. Treatments that inhibit REV7, she explains, could be used in tandem with frontline chemotherapies like cisplatin to prevent resistance.
Since her foray into biology at the American Museum of Natural History almost a decade ago, Vassel has maintained her passion for science outreach. During her time at MIT, she has served as a math tutor for middle schoolers in the Cambridge public school system. She also volunteered as a science and math mentor for high school students, as part of a dual athletic and academic program founded by MIT.
As Vassel wraps up her final year of graduate studies, she is torn between completing an academic postdoc and indulging her early interest in science education policy.
“Growing up in New York City, it was not lost on me that — despite the city’s wonderful diversity — people from historically underserved groups were still missing from many science-related positions,” Vassel says. “It got me thinking about the dire need for policymakers to improve curricula to make science more inclusive of all life experiences. There’s this idea that science is apolitical when it’s really not, and that mindset can have detrimental effects on equity and diversity in science.”