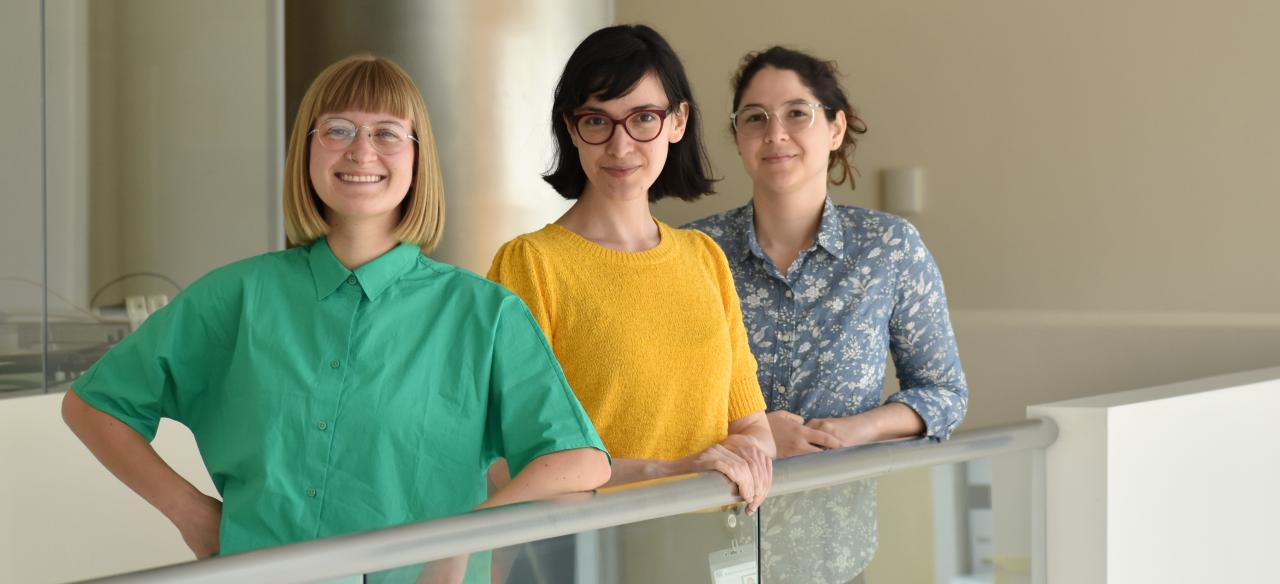
The paths three graduate students forged to the same Picower Institute lab illustrate the value of participating in the MIT Summer Research Program in Biology and Neuroscience.
David Orenstein | The Picower Institute for Learning and Memory
August 16, 2023
Doctoral studies at MIT aren’t a calling for everyone, but they can be for anyone who has had opportunities to discover that science and technology research is their passion and to build the experience and skills to succeed. For Taylor Baum, Josefina Correa Menéndez and Karla Alejandra Montejo, three graduate students in just one lab of The Picower Institute for Learning and Memory, a pivotal opportunity came via the MIT Summer Research Program in Biology and Neuroscience (MSRP BIO). When a student finds MSRP-BIO, it helps them find their future in research.
In the program undergraduate STEM majors from outside MIT spend the summer doing full-time research in the Departments of Biology or Brain and Cognitive Sciences (BCS), or the Center for Brains, Minds and Machines (CBMM). They gain lab skills, mentoring, preparation for graduate school and connections that might last a lifetime. Over the last two decades, a total of 215 students from under-represented minority groups, who are from economically-disadvantaged backgrounds, first-generation or non-traditional college students, or students with disabilities have participated in research in BCS or CBMM labs.
Like Baum, Correa Menéndez, and Montejo, the vast majority go on to pursue graduate studies, said Diversity & Outreach Coordinator Mandana Sassanfar, who runs the program. For instance, among 91 students who have worked in Picower Institute labs, 81 have completed their undergraduate studies. Of those, 46 enrolled in PhD programs at MIT or other schools such as Cornell, Yale, Stanford, Princeton, and the University of California System. Another 12 have gone to medical school, another 7 are in MD/PhD programs and 3 have earned master’s degrees. The rest are studying as post-baccalaureates or went straight into the workforce after earning their bachelor’s.
After participating in the program, Baum, Correa Menéndez, and Montejo each became graduate students in the research group of Emery N. Brown, Edward Hood Taplin Professor of Computational Neuroscience and Medical Engineering in The Picower Institute and the Institute for Medical Engineering and Science. The lab combines statistical, computational and experimental neuroscience methods to study how general anesthesia affects the central nervous system to ultimately improve patient care and advance understanding of the brain. Brown said the students have each been doing “off the scale” work, in keeping with the excellence he’s seen from MSRP BIO students over the years.
“I think MSRP is fantastic. Mandana does this amazing job of getting students who are quite talented to come to MIT to realize that they can move their game to the next level. They have the capacity to do it. They just need the opportunities,” Brown said. “These students live up to the expectations that you have of them. And now as graduate students, they’re taking on hard problems and they’re solving them.”
Paths to PhD studies
Pursuing a PhD is hardly a given. Many young students have never considered graduate school or specific fields of study like neuroscience or electrical engineering. But Sassanfar engages students across the country to introduce them to the opportunity MSRP BIO provides to gain exposure, experience and mentoring in advanced fields. Every fall, after the program’s students have returned to their undergraduate institutions, she visits schools in places as far flung as Florida, Maryland, Puerto Rico, and Texas and goes to conferences for diverse science communities such as ABRCMS and SACNAS to spread the word.
When Baum first connected with the program in 2017, she was finding her way at Penn State University. She had been majoring in biology and music composition but had just switched the latter to engineering following a conversation over coffee exposing her to brain-computer interfacing technology, in which detecting of brain signals of people with full-body paralysis could improve their quality of life by enabling control of computers or wheelchairs. Baum became enthusiastic about the potential to build similar systems, but as a new engineering student, she struggled to find summer internships and research opportunities.
“I got rejected from every single progam except the MIT Center for Brains Minds and Machines MSRP,” she recalled with a chuckle.
Baum thrived in MSRP BIO, working in Brown’s lab for three successive summers. At each stage, she said, she gained more research skills, experience and independence. When she graduated, she was sure she wanted to go to graduate school and applied to four of her dream schools. She accepted MIT’s offer to join the Department of Electrical Engineering and Computer Science, where she is co-advised by faculty members there and by Brown. She is now working to develop a system grounded in cardiovascular physiology that can improve blood pressure management. A tool for practicing anesthesiologists, the system automates the dosing of drugs to maintain a patient’s blood pressure at safe levels in the operating room or intensive care unit.
More than that, Baum not only is leading an organization advancing STEM education in Puerto Rico, but also is helping to mentor a current MSRP BIO student in the Brown lab.
“MSRP definitely bonds everyone who has participated in it,” Baum said. “If I see anyone who I know participated in MSRP, we could have an immediate conversation. I know that most of us, if we needed help, we’d feel comfortable asking for help from someone from MSRP. With that shared experience, we have a sense of camaraderie, and community.”
In fact, a few years ago when a former MSRP BIO student named Karla Montejo was applying to MIT, Baum provided essential advice and feedback about the application process, Montejo said. Now as a graduate student, Montejo has become a mentor for the program in her own right, Sassanfar noted. For instance, Montejo serves on program alumni panels that advise new MSRP BIO students.
Montejo’s family immigrated to Miami from Cuba when she was a child. The magnet high school she attended was so new that students were encouraged to help establish the school’s programs. She forged a path into research.
“I didn’t even know what research was,” she said. “I wanted to be a doctor, and I thought maybe it would help me on my resume. I thought it would be kind of like shadowing, but no, it was really different. So I got really captured by research when I was in high school.”
Despite continuing to pursue research in college at Florida International University, Montejo didn’t get into graduate school on her first attempt because she hadn’t yet learned how to focus her application. But Sassanfar had visited FIU to recruit students and through that relationship Montejo had already gone through MIT’s related Quantitative Methods Workshop (QMW). So Montejo enrolled in MSRP BIO, working in the CBMM-affiliated lab of Gabriel Kreiman at Boston Children’s Hospital.
“I feel like Mandana really helped me out gave me a break, and the MSRP experience pretty much solidified that I really wanted to come to MIT,” Montejo said.
In the QMW, Montejo learned she really liked computational neuroscience and in Kreiman’s lab she got to try her hand at computational modeling of the cognition involved in making perceptual sense of complex scenes. Montejo realized she wanted to work on more biologically based neuroscience problems. When the summer ended, because she was off the normal graduate school cycle for now, she found a two-year postbaccalaurate program at Mayo Clinic studying the role a brain cell type called astrocytes might have in the Parkinson’s Disease treatment deep brain stimulation.
When it came time to re-apply to graduate schools (with the help of Baum and others in the BCS Application Assistance Program) Montejo applied to MIT and got in, joining the Brown lab. Now she’s working on modeling the role of metabolic processes in the changing of brain rhythms under anesthesia, , taking advantage of how general anesthesia predictably changes brain states. The effects anesthetic drugs have on cell metabolism and the way that ultimately affects levels of consciousness reveals important aspects of how metabolism affects brain circuits and systems. Earlier this month, for instance, Montejo co-led a paper the lab published in The Proceedings of the National Academy of Sciences detailing the neuroscience of a patient’s transition into an especially deep state of unconsciousness called “burst suppression.”
A signature of the Brown lab’s work is rigorous statistical analysis and methods, for instance to discern brain arousals states from EEG measures of brain rhythms. A PhD candidate in MIT’s Interdisciplinary Doctoral Program in Statistics, Correa Menéndez is advancing the use of Bayesian hierarchical models for neural data analysis. These statistical models offer a principled way of pooling information across datasets. One of her models can help scientists better understand the way neurons can “spike” with electrical activity when the brain is presented with a stimulus. The other’s power is in discerning critical features such as arousal states of the brain under general anesthesia from electrophysiological recordings.
Though she now works with complex equations and computations as a PhD candidate in Neuroscience and Statistics, Correa Menéndez was mostly interested in music art as a high school student at Academia María Reina in San Juan and then architecture in college at the University of Puerto Rico, Río Piedras campus. It was discussions at the intersection of epistemology and art during an art theory class that inspired Correa Menéndez to switch her major to biology and to take computer science classes, too.
When Sassanfar visited Puerto Rico in 2017, a computer science professor (Dr. Patricia Ordóñez) suggested that Correa Menéndez apply for a chance to attend the QMW. She did and that led her to also participate in MSRP BIO in the lab of Sherman Fairchild Professor Matt Wilson (a faculty member in BCS, CBMM and The Picower Institute). She joined in the lab’s studies of how spatial memories are represented in the hippocampus and how the brain makes use of those memories to help understand the world around it. With mentoring from then-postdoc Carmen Varela (now a faculty member at Florida State University), the experience not only exposed her to neuroscience, but also, she gained skills and experience with lab experiments, building research tools, and conducting statistical analyses. She ended up working in the Wilson lab as a research scholar for a year and began her graduate studies in September 2018.
Classes she took with Brown as a research scholar inspired her to join his lab as a graduate student.
“Taking the classes with Emery and also doing experiments made me aware of the role of statistics in the scientific process: from the interpretation of results to the analysis and the design of experiments,” she said. “More often than not, in science, statistics becomes this sort of afterthought—this ‘annoying’ thing that people need to do to get their paper published. But statistics as a field is actually a lot more than that. It’s a way of thinking about data. Particularly, Bayesian modeling provides a principled inference framework for combining prior knowledge into a hypothesis that you can test with data.”
To be sure, no one starts out with such inspiration about scientific scholarship, but MSRP BIO helps students find that passion for research and the paths it opens up.