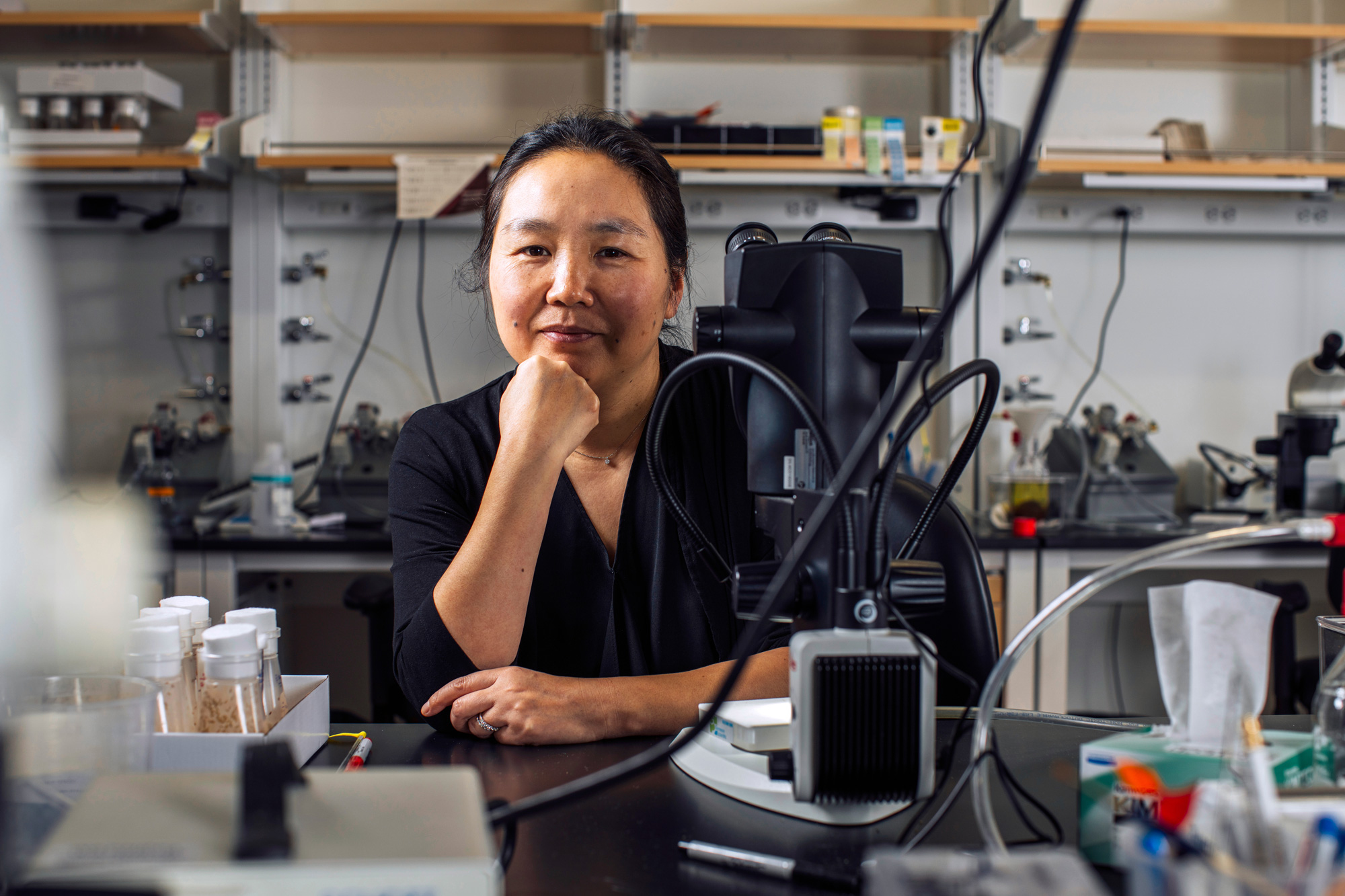
The MIT biologist’s research has shed light on the immortality of germline cells and the function of “junk DNA.”
Anne Trafton | MIT News Office
March 22, 2022
When cells divide, they usually generate two identical daughter cells. However, there are some important exceptions to this rule: When stem cells divide, they often produce one differentiated cell along with another stem cell, to maintain the pool of stem cells.
Yukiko Yamashita has spent much of her career exploring how these “asymmetrical” cell divisions occur. These processes are critically important not only for cells to develop into different types of tissue, but also for germline cells such as eggs and sperm to maintain their viability from generation to generation.
“We came from our parents’ germ cells, who used to be also single cells who came from the germ cells of their parents, who used to be single cells that came from their parents, and so on. That means our existence can be tracked through the history of multicellular life,” Yamashita says. “How germ cells manage to not go extinct, while our somatic cells cannot last that long, is a fascinating question.”
Yamashita, who began her faculty career at the University of Michigan, joined MIT and the Whitehead Institute in 2020, as the inaugural holder of the Susan Lindquist Chair for Women in Science and a professor in the Department of Biology. She was drawn to MIT, she says, by the eagerness to explore new ideas that she found among other scientists.
“When I visited MIT, I really enjoyed talking to people here,” she says. “They are very curious, and they are very open to unconventional ideas. I realized I would have a lot of fun if I came here.”
Exploring paradoxes
Before she even knew what a scientist was, Yamashita knew that she wanted to be one.
“My father was an admirer of Albert Einstein, so because of that, I grew up thinking that the pursuit of the truth is the best thing you could do with your life,” she recalls. “At the age of 2 or 3, I didn’t know there was such a thing as a professor, or such a thing as a scientist, but I thought doing science was probably the coolest thing I could do.”
Yamashita majored in biology at Kyoto University and then stayed to pursue her PhD, studying how cells make exact copies of themselves when they divide. As a postdoc at Stanford University, she became interested in the exceptions to that carefully orchestrated process, and began to study how cells undergo divisions that produce daughter cells that are not identical. This kind of asymmetric division is critical for multicellular organisms, which begin life as a single cell that eventually differentiates into many types of tissue.
Those studies led to a discovery that helped to overturn previous theories about the role of so-called junk DNA. These sequences, which make up most of the genome, were thought to be essentially useless because they don’t code for any proteins. To Yamashita, it seemed paradoxical that cells would carry so much DNA that wasn’t serving any purpose.
“I couldn’t really believe that huge amount of our DNA is junk, because every time a cell divides, it still has the burden of replicating that junk,” she says. “So, my lab started studying the function of that junk, and then we realized it is a really important part of the chromosome.”
In human cells, the genome is stored on 23 pairs of chromosomes. Keeping all of those chromosomes together is critical to cells’ ability to copy genes when they are needed. Over several years, Yamashita and her colleagues at the University of Michigan, and then at MIT, discovered that stretches of junk DNA act like bar codes, labeling each chromosome and helping them bind to proteins that bundle chromosomes together within the cell nucleus.
Without those barcodes, chromosomes scatter and start to leak out of the cell’s nucleus. Another intriguing observation regarding these stretches of junk DNA was that they have much greater variability between different species than protein-coding regions of DNA. By crossing two different species of fruit flies, Yamashita showed that in cells of the hybrid offspring flies, chromosomes leak out just as they would if they lost their barcodes, suggesting that the codes are specific to each species.
“We think that might be one of the big reasons why different species become incompatible, because they don’t have the right information to bundle all of their chromosomes together into one place,” Yamashita says.
Stem cell longevity
Yamashita’s interest in stem cells also led her to study how germline cells (the cells that give rise to eggs and sperm cells) maintain their viability so much longer than regular body cells across generations. In typical animal cells, one factor that contributes to age-related decline is loss of genetic sequences that encode genes that cells use continuously, such as genes for ribosomal RNAs.
A typical human cell may have hundreds of copies of these critical genes, but as cells age, they lose some of them. For germline cells, this can be detrimental because if the numbers get too low, the cells can no longer form viable daughter cells.
Yamashita and her colleagues found that germline cells overcome this by tearing sections of DNA out of one daughter cell during cell division and transferring them to the other daughter cell. That way, one daughter cell has the full complement of those genes restored, while the other cell is sacrificed.
That wasteful strategy would likely be too extravagant to work for all cells in the body, but for the small population of germline cells, the tradeoff is worthwhile, Yamashita says.
“If skin cells did that kind of thing, where every time you make one cell, you are essentially trashing the other one, you couldn’t afford it. You would be wasting too many resources,” she says. “Germ cells are not critical for viability of an organism. You have the luxury to put many resources into them but then let only half of the cells recover.”