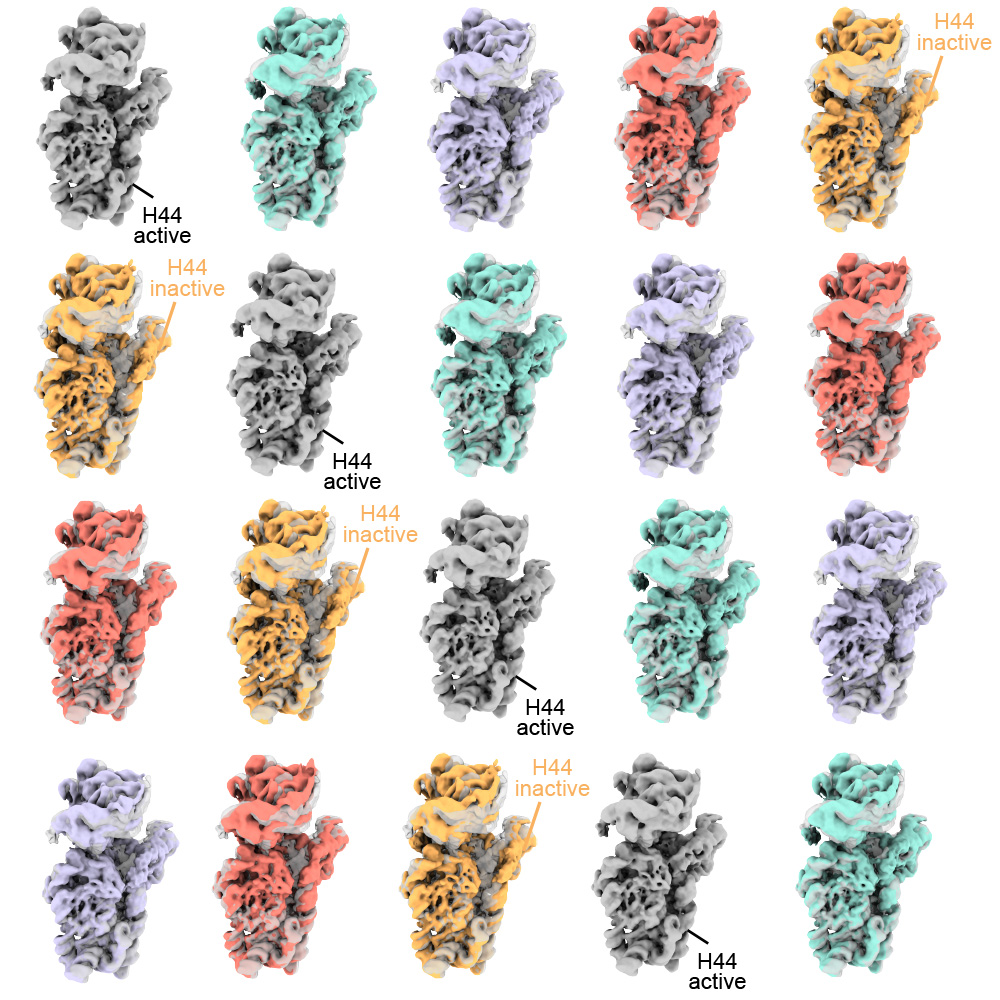
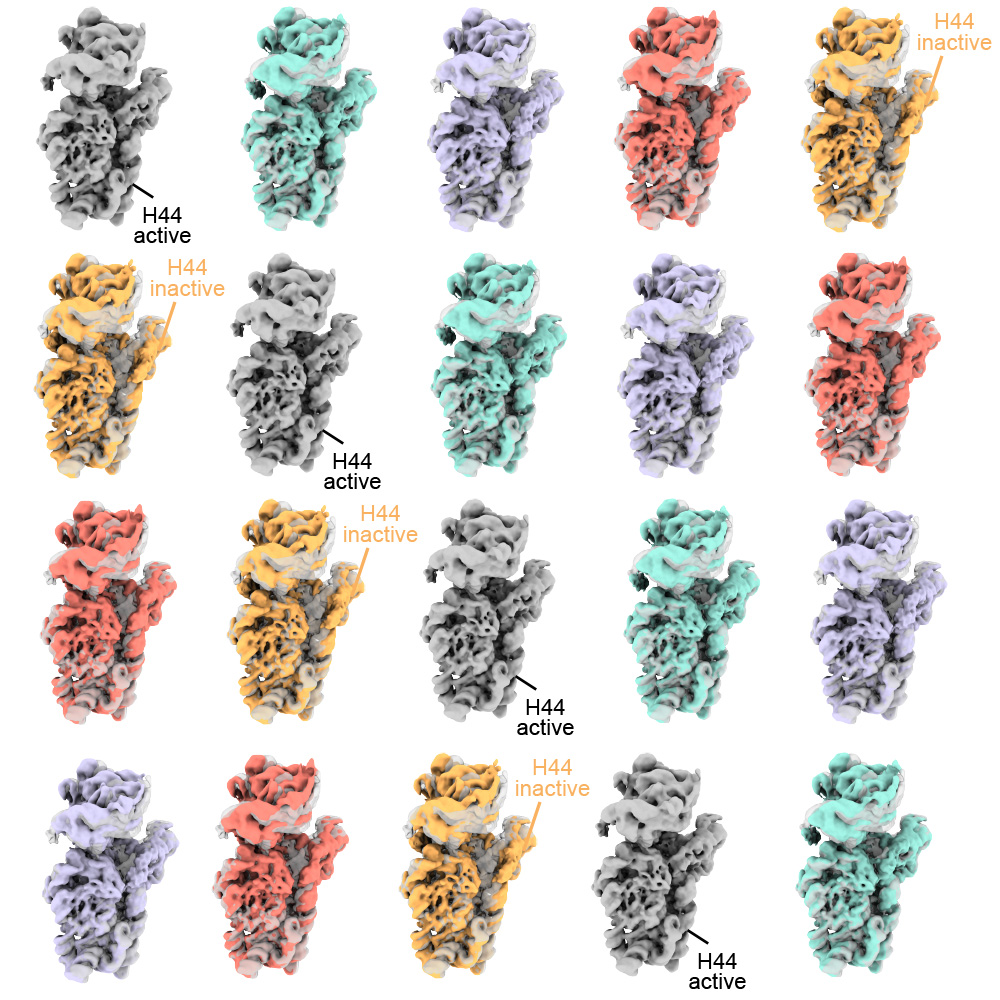
Sipping a beer on an early autumn evening, one might not consider that humans and yeast have been inextricably linked for thousands of years; winemaking, baking, and brewing all depend on budding yeast. Outside of baking and fermentation, researchers also use Saccharomyces cerevisiae, classified as a fungus, to study fundamental questions of cell biology.
Budding yeast gets its name from the way it multiplies. A daughter cell forms first as a swelling, protruding growth on the mother cell. The daughter cell projects further and further from the mother cell until it detaches as an independent yeast cell.
How do cells decide on a front and back? How do cells decode concentration gradients of chemical signals to orient in useful directions, or sense and navigate around physical obstacles? New Department of Biology faculty member Daniel “Danny” Lew uses the model yeast S. cerevisiae, and a non-model yeast with an unusual pattern of cell division, to explore these questions.
Q: Why is it useful to study yeast, and how do you approach the questions you hope to answer?
A: Humans and yeast are descended from a common ancestor, and some molecular mechanisms developed by that ancestor have been around for so long that yeast and mammals often use the same mechanisms. Many cells develop a front and migrate or grow in a particular direction, like the axons in our nervous system, using similar molecular mechanisms to those of yeast cells orienting growth towards the bud.
When I started my lab, I was working on cell cycle control, but I’ve always been interested in morphogenesis and the cell biology of how cells change shape and decide to do different things with different parts of themselves. Those mechanisms turn out to be conserved between yeast and humans.
But some things are very different about fungal and animal cells. One of the differences is the cell wall and what fungal cells have to do to deal with the fact that they have a cell wall.
Fungi are inflated by turgor pressure, which pushes their membranes against the rigid cell wall. This means they’ll die if there is any hole in the cell wall, which would be expected to happen often as cells remodel the wall in order to grow. We’re interested in understanding how fungi sense when any weak spots appear in the wall and repair them before those weak spots become dangerous.
Yeast cells, like most fungi, also mate by fusing with a partner. To succeed, they must do the most dangerous thing in the fungal life cycle: get rid of the cell wall at the point of contact to allow fusion. That means they must be precise about where and when they remove the wall. We’re fascinated to understand how they know it is safe to remove the wall there, and nowhere else.
We take an interdisciplinary approach. We’ve used genetics, biochemistry, cell biology, and computational biology to try and solve problems in the past. There’s a natural progression: observation and genetic approaches tend to be the first line of attack when you know nothing about how something works. As you learn more, you need biochemical approaches and, eventually, computational approaches to understand exactly what mechanism you’re looking at.
I’m also passionate about mentoring, and I love working with trainees and getting them fascinated by the same problems that fascinate me. I’m looking to work with curious trainees who love addressing fundamental problems.
Q: How does yeast decide to orient a certain way — toward a mating partner, for example?
A: We are still working on questions of how cells analyze the surrounding environment to pick a direction. Yeast cells have receptors that sense pheromones that a mating partner releases. What is amazing about that is that these cells are incredibly small, and pheromones are released by several potential partners in the neighborhood. That means yeast cells must interpret a very confusing landscape of pheromone concentrations. It’s not apparent how they manage to orient accurately toward a single partner.
That got me interested in related questions. Suppose the cell is oriented toward something that isn’t a mating partner. The cell seems to recognize that there’s an obstacle in the way, and it can change direction to go around that obstacle. This is how fungi get so good at growing into things that look very solid, like wood, and some fungi can even penetrate Kevlar vests.
If they recognize an obstacle, they have to change directions and go around it. If they recognize a mating partner, they have to stick with that direction and allow the cell wall to get degraded. How do they know they’ve hit an obstacle? How do they know a mating partner is different from an obstacle? These are the questions we’d like to understand.
Q: For the last couple of years, you’ve also been studying a budding yeast that forms multiple buds when it reproduces instead of just one. How did you come across it, and what questions are you hoping to explore?
A: I spent several years trying to figure out why most yeasts make one bud and only one bud, which I think is related to the question of why migrating cells make one and only one front. We had what we thought was a persuasive answer to that, so seeing a yeast completely disobey that and make as many buds as it felt like was a shock, which got me intrigued.
We started working on it because my colleague, Amy Gladfelter, had sampled the waters around Woods Hole, Massachusetts. When she saw this specimen under a microscope, she immediately called me and said, “You have to look at this.”
A question we’re very intrigued by is if the cell makes five, seven, or 12 buds simultaneously, how do they divide the mother cell’s material and growth capacity five, seven, or 12 ways? It looks like all of the buds grow at the same rate and reach about the same size. One of our short-term goals is to check whether all the buds really get to exactly the same size or whether they are born unequal.
And we’re interested in more than just growth rate. What about organelles? Do you give each bud the same number of mitochondria, nuclei, peroxisomes, and vacuoles? That question will inevitably lead to follow-up questions. If each bud has the same number of mitochondria, how does the cell measure mitochondrial inheritance to do that? If they don’t have the same amount, then buds are each born with a different complement and ratio of organelles. What happens to buds if they have very different numbers of organelles?
As far as we can tell, every bud gets at least one nucleus. How the cell ensures that each bud gets a nucleus is a question we’d also very much like to understand.
We have molecular candidates because we know a lot about how model yeasts deliver nuclei, organelles, and growth materials from the mother to the single bud. We can mutate candidate genes and see if similar molecular pathways are involved in the multi-budding yeast and, if so, how they are working.
It turns out that this unconventional yeast has yet to be studied from the point of view of basic cell biology. The other thing that intrigues me is that it’s a poly-extremophile. This yeast can survive under many rather harsh conditions: it’s been isolated in Antarctica, from jet engines, from all kinds of plants, and of course from the ocean as well. An advantage of working with something so ubiquitous is we already know it’s not toxic to us under almost any circumstances. We come into contact with it all the time. If we learn enough about its cell biology to begin to manipulate it, then there are many potential applications, from human health to agriculture.
There were early signs that Nicole De Nisco, SB ‘07, PhD ‘13, might become a scientist. She ran out of science classes to take in high school and fondly remembers the teacher that encouraged her to pursue science instead of the humanities. But she ended up at MIT, in part, out of spite.
“I applied because my guidance counselor told me I wouldn’t get in,” she said. The rest, as they say, is history for the first-generation college student from Los Angeles.
Now, she’s an assistant professor of biological sciences at UT Dallas studying urinary tract infections (UTIs) and the urinary microbiome in postmenopausal women.
De Nisco has already made some important advancements in the field: she developed a new technique for visualizing bacteria in the bladder and used it to demonstrate that bacteria form reservoirs in human bladder tissue, leading to chronic or recurrent UTIs.
It was known that in mice, bacteria are able to create communities within the bladder tissue, forming reservoirs and staying there long term—but no one had shown that occurring in human tissue before.
De Nisco found that reservoirs of tissue-resident bacteria exist in human patients with recurring UTIs, a condition which may ultimately lead to women needing to have their bladder removed. De Nisco now mostly works with postmenopausal women who have been suffering from decades of recurring UTIs.
There was a big gap in the field, De Nisco explained, so entering the field of urology was also an opportunity to make new discoveries and find new ways to treat those recurring infections.
De Nisco said she’s in the minority, both as a woman studying urology and as someone studying diseases that affect female patients. Most researchers in the urology field are men, and most focus on the prostate.
But things are changing.
“I think there are a lot of women in the field who are now pushing back, and I actually collaborate with a lot of other female investigators in the field. We’re trying to support each other so that we can survive and, hopefully, actually advance the science—instead of it being in the same place it was 15 years ago,” De Nisco says.
De Nisco first fell in love with biomedical research as an undergrad doing a UROP in Catherine Drennan’s lab, back when Drennan was still located in the chemistry building.
“Cathy herself was incredibly encouraging, and is probably the main reason I decided to pursue a career in science—or felt that I could,” De Nisco said.
De Nisco became fascinated with the dialogue between a microbe and a host organism during an undergraduate course in microbial physiology with Graham Walker, which led to De Nisco’s decision to remain at MIT for her PhD work and to perform her doctoral research in rhizobia legume symbiosis in Walker’s lab.
De Nisco said during her time at MIT, Drennan and Walker gave her a lot of encouragement and “room to do my own thing,” fostering a love of discovery and problem solving. It’s a mentoring style she’s using now with her own graduate students; she currently has eight working in her lab.
“Every student is different: some just want a project and they want to know what they’re doing, and some want to explore,” she said. “I was the type that wanted to do my own thing and so they gave me the room and the patience to be able to explore and find something new that I was interested in and excited about.”
As a low-income student sending financial help home, she also pursued teaching opportunities outside of her usual duties; Walker was very supportive of pursuing other teaching opportunities. De Nisco was a graduate student tutor for Next House watching over 40 undergrads, served as a teaching fellow with the Harvard Extension School, and worked with Eric Lander to help launch the course 7.00x Introduction to Biology – The Secret of Life for EdX, one of the most highly rated MOOCs of all time.
She said MIT definitely prepared her for a life as a professor, teacher, and mentor; the most important thing about graduate school isn’t choosing “the most cutting-edge research project,” but making sure you have a good training experience and an advisor who can provide that.
“You don’t need to start building your name in the field when you’re a grad student. The lab environment is much more important than the topic. It’s easy to get burned out or to be turned off to a career in academia altogether if you have the wrong advisor,” she said. “You need to learn how to be a scientist, and you have plenty of time later in your career to follow whatever path you want to follow.”
She knows this from experience: her current research is somewhat parallel but unrelated to her previous research experience.
“I think my motivation for being a scientist is rooted in my desire to help people doing something I enjoy,” she said. “I was not doing this kind of research as a graduate student, and that doesn’t mean that I wasn’t able to end up at this point in my career where I’m doing research that is focused on improving the lives of women, specifically.”
She did her postdoctoral work at UT Southwestern Medical Center studying Vibrio parahaemolyticus, a human pathogen that causes gastroenteritis. The work was a marriage of her interests in biochemistry and host-microbiome interactions.
She said MIT prepared her well for the type of interdisciplinary work that she does every day: At UT Dallas, all the research buildings are fully integrated, with engineers, chemists, physicists, and biologists sharing lab spaces in the same building. Her closest collaborators are mathematicians, chemists, and engineers.
Although she may not be fully literate, she has a common language with the people she works with thanks to MIT’s undergraduate course requirements in many different topics and MIT’s focus on interdisciplinary research, which is “how real advancement is made.”
Ultimately, De Nisco said she is glad to this day that she attended MIT.
“Getting that acceptance letter to attend MIT probably changed the trajectory of my life,” she said. “You never know, on paper, what someone is going to achieve eventually, and what kind of force they’re going to be. I’m always grateful to whoever was on the admissions committees that made the decision to accept me—twice.”
Your mouth is a crucial interface between the outside world and the inside of your body. Everything you breathe, chew or drink interacts with your oral cavity—the proteins and the microbes, including microbes that can harm us. When things go awry, the result can range from the mild, like bad breath, to the serious, like tooth and gum decay to more dire effects in the gut and other parts of the body.
Even though the oral microbiome plays a critical role as a front-line defense for human health and disease, we still know very little about the intricacies of host-microbe interactions in the complex physiological environment of the mouth; a better understanding of those interactions is key to developing treatments for human disease.
In a recent study published in PNAS, a collaborative effort revealed that one of the most abundant proteins found in our saliva binds to the surface of select microbes found in the mouth. The findings shed light on how salivary proteins and mucus play a role in maintaining the oral cavity microbiome.
The collaboration involved members of the Imperiali lab in the Department of Biology and the Kiessling lab in the Department of Chemistry at MIT, as well as the Ruhl group at the University at Buffalo School of Dental Medicine, and the Grimes group at the University of Delaware.
The paper is focused on an abundant oral cavity protein called zymogen granule protein 16 homolog B (ZG16B). Finding ZG16B’s interaction partners and gaining insight into its function were the overarching goals of the project. To accomplish this, Ghosh and colleagues engineered ZG16B to add reporter tags such as fluorophores. They called these modified proteins “microbial glycan analysis probes (mGAPs)” because they allowed them to identify ZG16B binding partners using complementary methods. They applied the probes to samples of healthy oral microbiomes to identify target microbes and binding partners.
The results excited them.
“ZG16B didn’t just bind to random bacteria. It was very focused on certain species including a commensal bacteria called Streptococcus vestibularis,” says first author Soumi Ghosh, a postdoctoral associate in the Imperiali lab.
Commensal bacteria are found in a normal healthy microbiome and do not cause disease.
Using the mGAPs, the team showed that ZG16B binds to cell wall polysaccharides of the bacteria, which indicates that ZG16B is a lectin, a carbohydrate-binding protein. In general, lectins are responsible for cell-cell interactions, signaling pathways, and some innate immune responses against pathogens. “This is the first time that it has been proven experimentally that ZG16B acts as a lectin because it binds to the carbohydrates on the cell surface or cell wall of the bacteria,” Ghosh highlights.
ZG16B was also shown to recruit Mucin 7 (MUC7), a salivary glycoprotein in the oral cavity, and, together the results suggest that ZG16B may help maintain a healthy balance in the oral microbiome by forming a complex with MUC7 and certain bacteria. The results indicate that ZG16B regulates the bacteria’s abundance by preventing overgrowth through agglutination when the bacteria exceed a certain level of growth.
“ZG16B, therefore, seems to function as a missing link in the system; it binds to different types of glycans—the microbial glycans and the mucin glycans—and ultimately, maintains a healthy balance in our oral cavity,” Ghosh says.
Further work with this probe and samples of oral microbiome from healthy and diseased subjects could also reveal the lectin’s importance for oral health and disease.
Current attention is focused on developing and applying additional mGAPs based on other human lectins, such as those found in serum, liver, and intestine to reveal their binding specificities and their roles in host-microbe interactions.
“The research carried out in this collaboration exemplifies the kind of synergy that made me excited to move to MIT 5 years ago,” says senior co-author Laura Kiessling. “I’ve been able to work with outstanding scientists who share my interest in the chemistry and the biology of carbohydrates.”
The senior authors of the paper—Barbara Imperiali and Kiessling — came up with the term for the probes they’re creating: “mGAPS to fill in the gaps” in our understanding of the role of lectins in the human microbiome, according to Ghosh.
“If we want to develop therapeutics against bacterial infection, we need a better understanding of host-microbe interactions,” Ghosh says. “The significance of our study is to prove that we can make very good probes for microbial glycans, find out their importance in the frontline defense of the immune system, and, ultimately, come up with a therapeutic approach to disease.”
This research was supported by the National Institute of Health.
SARS-CoV-2, the virus that causes COVID-19, seems to have become a permanent presence in our lives. Research from Whitehead Institute Founding Member Rudolf Jaenisch’s lab reveals that this may be true on multiple levels. Jaenisch, postdoc Liguo Zhang, and colleagues have shown that when the virus infects people, it is capable of integrating parts of its genetic code into the human genome through a process called reverse transcription. This genomic integration is rare, but due to how many hundreds of millions of people have been infected, it has likely occurred many times.
In a paper published in the journal Viruses on February 25, the researchers use and compare multiple methods to show that SARS-CoV-2 can integrate into host cells’ genomes. The paper is a follow up to Jaenisch and Zhang’s 2021 paper in the Proceedings of the National Academy of Sciences, which provided initial evidence of SARS-CoV-2 genomic integration. The original paper intended to solve the puzzle of why some people who had had COVID-19 were still testing positive long after recovering from the disease. The answer the researchers found was that parts of the viral genome were reverse transcribed into the human genome, meaning the viral RNA was transcribed or “read” into DNA (a reverse of the usual process) and then that DNA was stitched into the cell’s DNA. Then, when the cells’ genomes were transcribed into RNA, the portion of the virus’ genome that had been incorporated would be included and could be recognized by a PCR test, leading to a positive result.
In order to further substantiate the findings described in the previous paper, Jaenisch and Zhang have now performed additional experiments and analyses. The new paper explains why some experiments testing for viral genomic integration would come up with a negative result, and how this is consistent with Jaenisch and Zhang’s conclusion. Additionally, Jaenisch and Zhang examine whether viral RNA put into cells, as a model of the COVID-19 mRNA vaccines, can also integrate into the human genome, and find initial evidence that it cannot.
“This paper puts our data on a very firm footing,” Jaenisch says. “Hopefully, it will clarify some of the issues raised in the discussion that followed the first paper, and provide some reassurance to people who were worried about the implications for the vaccine.”
The main challenge in finding evidence of SARS-CoV-2 integrating into the human genome is that this event appears to be very rare. In the new paper, Jaenisch and Zhang used digital PCR, an approach that can sensitively detect specific DNA sequences in cells, to see how commonly the sequence that they would find in instances of viral RNA being read into DNA appeared in infected cells. Specifically, they looked for reverse transcribed SARS-CoV-2 complementary DNA (cDNA), DNA that is made from the virus’ original mRNA. Digital PCR revealed that for every one thousand cells, reverse transcribed viral cDNA was only present in around four to twenty cells. This number includes all detected instances of viral cDNA, whether integrated into the genome or not, so genomic integration is likely even rarer—indeed, the new research suggests that only a fraction of the total cDNA identified is from genomic integration.
Because genomic viral integration is so rare, Jaenisch and Zhang needed to use multiple complementary methods to test for it. One approach, called whole genome sequencing (WGS), is able to search cells’ genomes in great detail. When it does come across an instance of viral genomic integration, it can identify not only the reverse transcribed viral sequence, but also two sequences near the viral sequence that are added when it is integrated into the genome by a common reverse transcription complex called LINE1, which is encoded in the host cells. The combination of viral cDNA plus the two nearby cellular host sequences provides very strong evidence that viral cDNA is not only present but has been incorporated into the cell’s genome. However, WGS can only search the equivalent of a few cells’ genomes, and so when searching for a rare event, like SARS-CoV-2 integration, it often comes up empty. People skeptical of the first paper performed this type of experiment and came up with a negative result; Jaenisch and Zhang were not surprised by that, and it is consistent with their own findings when using this approach.
“Because the human cell genome coverage by whole genome sequencing is very limited, you would need to run the sequencing experiment many times in order to have a good chance of detecting one viral genome copy,” Zhang says.
In order to make the most of WGS, Jaenisch and Zhang induced their cells to overexpress LINE1, the cellular machinery that reverse transcribes viral RNA into the human genome. This exponentially increases the amount of viral cDNA that gets made; when the researchers performed digital PCR on their cells with overexpression, it detected fourteen to twenty thousand cDNA copies per thousand cells. Consequently, WGS was able to detect instances of viral cDNA plus the two nearby sequences that are the telltale signature of genomic integration in these cells.
“This is unambiguous proof of viral genomic integration,” Zhang says.
This type of experiment is called a positive control. Researchers use it to prove that, in ideal circumstances, the biological phenomenon they are curious about can occur. The question then becomes: does the phenomenon happen in normal circumstances? This was a criticism raised by some researchers in response to the first paper: they were not convinced that viral genomic integration happens in the cells of an infected person, which do not have the same levels of LINE1.
Jaenisch and Zhang used another approach to hunt for evidence of viral genomic integration in cells without LINE1 overexpression. The approach, called an enrichment method and performed with the tool TagMap, can analyze thousands of cells—enough cells to reliably find evidence of a rare event. However, it cannot get the same detail as whole genome sequencing; TagMap enriches and captures shorter sequences of DNA, so it can only capture one of the two nearby sequences that act as a signature alongside viral cDNA. However, the smaller stretch of DNA that the researchers focused on still has features that can be used as evidence of integration. With this approach, Jaenisch and Zhang detected many instances of viral cDNA linked to the nearby cellular sequence.
Jaenisch and Zhang argue that the combined results of these experiments show strong proof of viral integration. Whole genome sequencing provides very strong proof that viral genomic integration can occur in the right conditions. Enrichment with TagMap provides reasonably strong proof that viral genomic integration occurs in normal cells.
“Each of these methods has advantages and disadvantages. You have to combine them to get the complete picture,” Jaenisch says.
After reaffirming their results that genomic integration of SARS-CoV-2 happens following viral infection, the researchers wanted to know whether the same thing happens with mRNA from the COVID-19 vaccines—which had been a concern expressed by many in the wake of the first paper. Jaenisch and Zhang could not get access to the actual vaccine RNA, packaged into a lipid coat, which is used for vaccination. Instead, they created a model of vaccine injection, inserting a bit of SARS-CoV-2 genetic material (mRNA) into cells through transfection, or non-infection “delivery” of genetic content into cells.
The researchers found that transfection of SARS-CoV-2 mRNA did not lead to genomic integration in the same way that infection did. Infection naturally produces a large amount of viral RNA and causes an inflammatory response in cells. Such cellular stresses increase the level of the reverse transcription machinery. Transfection does not do this, and correspondingly, the researchers found no evidence with TagMap that it led to viral genomic integration by LINE1 in normal cells.
The researchers’ model of vaccine injection is missing several key features of the actual vaccine. In the future, Jaenisch hopes to follow up on this research using the actual vaccine RNA sequence, and testing in an animal model to more closely match what happens during vaccine injection. In the meantime, the researchers hope that these initial results are reassuring.
“We need to do further testing, but our results are consistent with vaccine RNA not integrating,” Jaenisch says.
Zhang, Liguo, Punam Bisht, Anthony Flamier, M. Inmaculada Barrasa, Max Friesen, Alexsia Richards, Stephen H. Hughes, and Rudolf Jaenisch. 2023. “LINE1-Mediated Reverse Transcription and Genomic Integration of SARS-CoV-2 mRNA Detected in Virus-Infected but Not in Viral mRNA-Transfected Cells” Viruses 15, no. 3: 629. https://doi.org/10.3390/v15030629
Different cells take on an astonishing variety of shapes, which are often critical to be able to perform specialized cell functions like absorbing nutrients or contracting muscles. We study how different cell shapes arise and how cells control the spatial distribution of their internal constituents. We take advantage of the tractability of fungal model systems, and address these questions using approaches from cell biology, genetics, and computational biology to understand molecular mechanisms.
From the tropics to the poles, from the sea surface to hundreds of feet below, the world’s oceans are teeming with one of the tiniest of organisms: a type of bacteria called Prochlorococcus, which despite their minute size are collectively responsible for a sizable portion of the oceans’ oxygen production. But the remarkable ability of these diminutive organisms to diversify and adapt to such profoundly different environments has remained something of a mystery.
Now, new research reveals that these tiny bacteria exchange genetic information with one another, even when widely separated, by a previously undocumented mechanism. This enables them to transmit whole blocks of genes, such as those conferring the ability to metabolize a particular kind of nutrient or to defend themselves from viruses, even in regions where their population in the water is relatively sparse.
The findings describe a new class of genetic agents involved in horizontal gene transfer, in which genetic information is passed directly between organisms — whether of the same or different species — through means other than lineal descent. The researchers have dubbed the agents that carry out this transfer “tycheposons,” which are sequences of DNA that can include several entire genes as well as surrounding sequences, and can spontaneously separate out from the surrounding DNA. Then, they can be transported to other organisms by one or another possible carrier system including tiny bubbles known as vesicles that cells can produce from their own membranes.
The research, which included studying hundreds of Prochlorococcus genomes from different ecosystems around the world, as well as lab-grown samples of different variants, and even evolutionary processes carried out and observed in the lab, is reported today in the journal Cell, in a paper by former MIT postdocs Thomas Hackl and Raphaël Laurenceau, visiting postdoc Markus Ankenbrand, Institute Professor Sallie “Penny” Chisholm, and 16 others at MIT and other institutions.
Chisholm, who played a role in the discovery of these ubiquitous organisms in 1988, says of the new findings, “We’re very excited about it because it’s a new horizontal gene-transfer agent for bacteria, and it explains a lot of the patterns that we see in Prochlorococcus in the wild, the incredible diversity.” Now thought to be the world’s most abundant photosynthetic organism, the tiny variants of what are known as cyanobacteria are also the smallest of all photosynthesizers.
Hackl, who is now at the University of Groningen in the Netherlands, says the work began by studying the 623 reported genome sequences of different species of Prochlorococcus from different regions, trying to figure out how they were able to so readily lose or gain particular functions despite their apparent lack of any of the known systems that promote/boost horizontal gene transfer, such as plasmids or viruses known as prophages.
What Hackl, Laurenceau, and Ankenbrand investigated were “islands” of genetic material that seemed to be hotspots of variability and often contained genes that were associated with known key survival processes such as the ability to assimilate essential, and often limiting, nutrients such as iron, or nitrogen, or phosphates. These islands contained genes that varied enormously between different species, but they always occurred in the same parts of the genome and sometimes were nearly identical even in widely different species — a strong indicator of horizontal transfer.
But the genomes showed none of the usual features associated with what are known as mobile genetic elements, so initially this remained a puzzle. It gradually became apparent that this system of gene transfer and diversification was different from any of the several other mechanisms that have been observed in other organisms, including in humans.
Hackl describes what they found as being something like a genetic LEGO set, with chunks of DNA bundled together in ways that could almost instantly confer the ability to adapt to a particular environment. For example, a species limited by the availability of particular nutrients could acquire genes necessary to enhance the uptake of that nutrient.
The microbes appear to use a variety of mechanisms to transport these tycheposons (a name derived from the name of the Greek goddess Tyche, daughter of Oceanus). One is the use of membrane vesicles, little bubbles pouched off from the surface of a bacterial cell and released with tycheposons inside it. Another is by “hijacking” virus or phage infections and allowing them to carry the tycheposons along with their own infectious particles, called capsids. These are efficient solutions, Hackl says, “because in the open ocean, these cells rarely have cell-to-cell contacts, so it’s difficult for them to exchange genetic information without a vehicle.”
And sure enough, when capsids or vesicles collected from the open ocean were studied, “they’re actually quite enriched” in these genetic elements, Hackl says. The packets of useful genetic coding are “actually swimming around in these extracellular particles and potentially being able to be taken up by other cells.”
Chisholm says that “in the world of genomics, there’s a lot of different types of these elements” — sequences of DNA that are capable of being transferred from one genome to another. However, “this is a new type,” she says. Hackl adds that “it’s a distinct family of mobile genetic elements. It has similarities to others, but no really tight connections to any of them.”
While this study was specific to Prochlorococcus, Hackl says the team believes the phenomenon may be more generalized. They have already found similar genetic elements in other, unrelated marine bacteria, but have not yet analyzed these samples in detail. “Analogous elements have been described in other bacteria, and we now think that they may function similarly,” he says.
“It’s kind of a plug-and-play mechanism, where you can have pieces that you can play around with and make all these different combinations,” he says. “And with the enormous population size of Prochlorococcus, it can play around a lot, and try a lot of different combinations.”
Nathan Ahlgren, an assistant professor of biology at Clark University who was not associated with this research, says “The discovery of tycheposons is important and exciting because it provides a new mechanistic understanding of how Prochlorococcus are able to swap in and out new genes, and thus ecologically important traits. Tycheposons provide a new mechanistic explanation for how it’s done.” He says “they took a creative way to fish out and characterize these new genetic elements ‘hiding’ in the genomes of Prochlorococcus.”
He adds that genomic islands, the portions of the genome where these tycheposons were found, “are found in many bacteria, not just marine bacteria, so future work on tycheposons has wider implications for our understanding of the evolution of bacterial genomes.”
The team included researchers at MIT’s Department of Civil and Environmental Engineering, the University of Wuerzburg in Germany, the University of Hawaii at Manoa, Ohio State University, Oxford Nanopore Technologies in California, Bigelow Laboratory for Ocean Sciences in Maine, and Wellesley College. The work was supported by the Simons Foundation, the Gordon and Betty Moore Foundation, the U.S. Department of Energy, and the U.S. National Science Foundation.