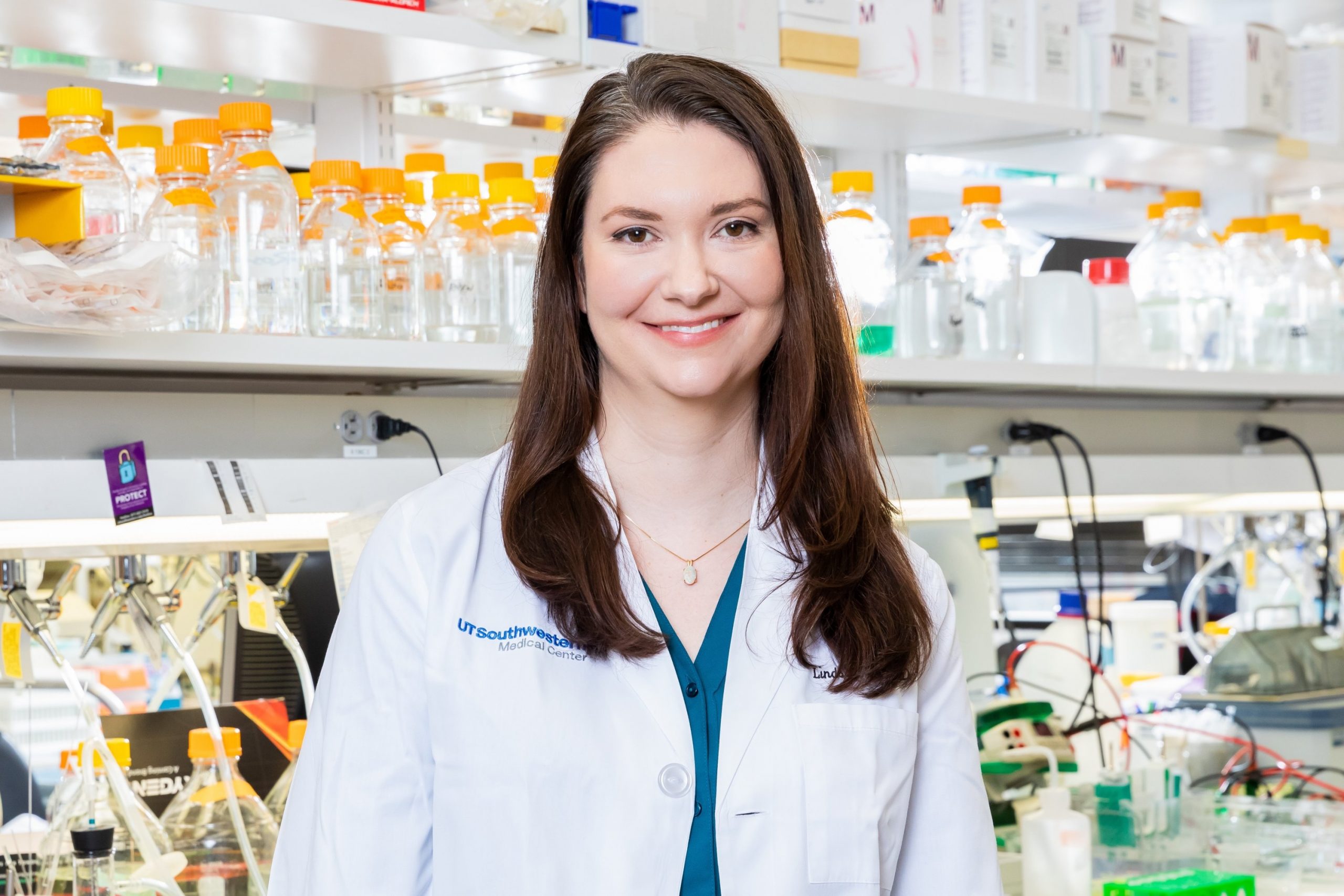
Case’s new lab investigates why cancer arises when disruptions in cellular organization change how cells sense mechanical forces.
Saima Sidik | Department of Biology
February 2, 2021
Assistant professor of biology Lindsay Case wants to understand the protein complexes called focal adhesions that let cells move and sense the world around them. She also aims to determine how cancer arises when focal adhesions malfunction. During her postdoc work at the University of Texas Southwestern Medical Center, she discovered that some of the proteins in focal adhesions work together because of phase separation — a clumping behavior that researchers are just beginning to understand. She sat down to discuss what her work means for cancer research, and her future plans for her new lab in the Department of Biology.
Q: What is phase separation, and how does it affect the way cells function?
A: I always compare phase separation to the separation of oil and vinegar. Something similar can happen with almost any kind of molecule, including proteins. When the interactions between proteins are stronger than their interactions with their surroundings, they can segregate into something called a liquid phase, similar to oil droplets floating in vinegar.
Phase separation matters because organization is a major challenge for our cells, which contain tons of different types of molecules. Sometimes cells organize these molecules using membranes, which is like using fences to keep them in place. But many subcellular structures aren’t surrounded by membranes, and how these compartments keep their components together has been a big mystery since scientists first observed them under microscopes in the 1800s. It’s really only in the last 10 years that people have realized that phase separation is part of the answer.
When cells lose the ability to stay organized, it can have devastating consequences. Changes in how proteins phase separate might underlie serious diseases, like Alzheimer’s and ALS [amyotrophic lateral sclerosis]. I’m really interested in how phase separation organizes protein complexes called focal adhesions, which link cells to their external environment. One function of focal adhesion is to let cells sense mechanical forces from the outside world, and when cells lose this ability it can contribute to cancer progression.
Q: How did phase separation initially pique your interest, and how has your research career prepared you for the work you’ll do at MIT?
A: During my PhD, I was studying how molecules within focal adhesions are organized. I saw a talk by Michael Rosen from the University of Texas Southwestern Medical Center, who would later become my postdoc advisor. Phase separation of proteins was a new idea at the time, but Mike thought it was a powerful force underlying protein organization that we needed to understand more thoroughly. I was intrigued because, at the time, I was unsure what drove focal adhesions to assemble on the plasma membrane, and I wondered if that arrangement might be due to phase separation.
I ended up joining Mike’s lab for my postdoc so that I could apply his ideas about phase separation to my interest in cell signaling and focal adhesions. As a result, I ended up working in a field as it was being born. The first year of my postdoc there were only a few papers investigating phase separation in cellular organization, and now there are over 1,000. Seeing this rapid progress firsthand has been exciting. One of the highlights of my postdoc was showing that phase separation can actually affect the functions of signaling proteins organized on membranes, and I think this discovery went a long way towards showing that phase separation isn’t just a thing that cells can do — it’s something they need to do to survive.
MIT will be an awesome place to continue studying how phase separation lets cells sense the world around them. It’s one of the institutes where the idea of phase separation in biology took off, and the MIT scientists who work on phase separation come from so many different research backgrounds. Understanding phase separation is going to require an interdisciplinary approach, which MIT values. Plus, the students are amazing!
Q: What makes your approach to studying cancer unique?
A: A lot of cancer researchers focus on large-scale or small-scale aspects of these diseases. They either look at how cancer cells behave as a whole, or study the behavior of just one protein. But there’s a level in between where I want to focus my work. I can figure out how large, multi-protein complexes like focal adhesions — some of which form because of phase separation — affect disease progression. During my postdoc, I developed a way to recreate simplified focal adhesions outside of cells. I want to use this system to learn more about how phase separation lets these complexes sense mechanical forces, and how this changes in cancer cells.
Some of the proteins found in focal adhesions are tethered to the plasma membrane, and not many people have studied how protein phase separation changes when you throw a membrane into the mix. I’m excited to keep building up my simplified focal adhesion system in my new lab, and eventually recreate the rest of the complex.
As my lab becomes more established, I’d also like to study how phase separation affects interactions between different protein complexes and signaling pathways. Phase separation is such a rapidly evolving field that it’s hard to know where my research will lead, but that’s part of the fun — not knowing where my work will take me.