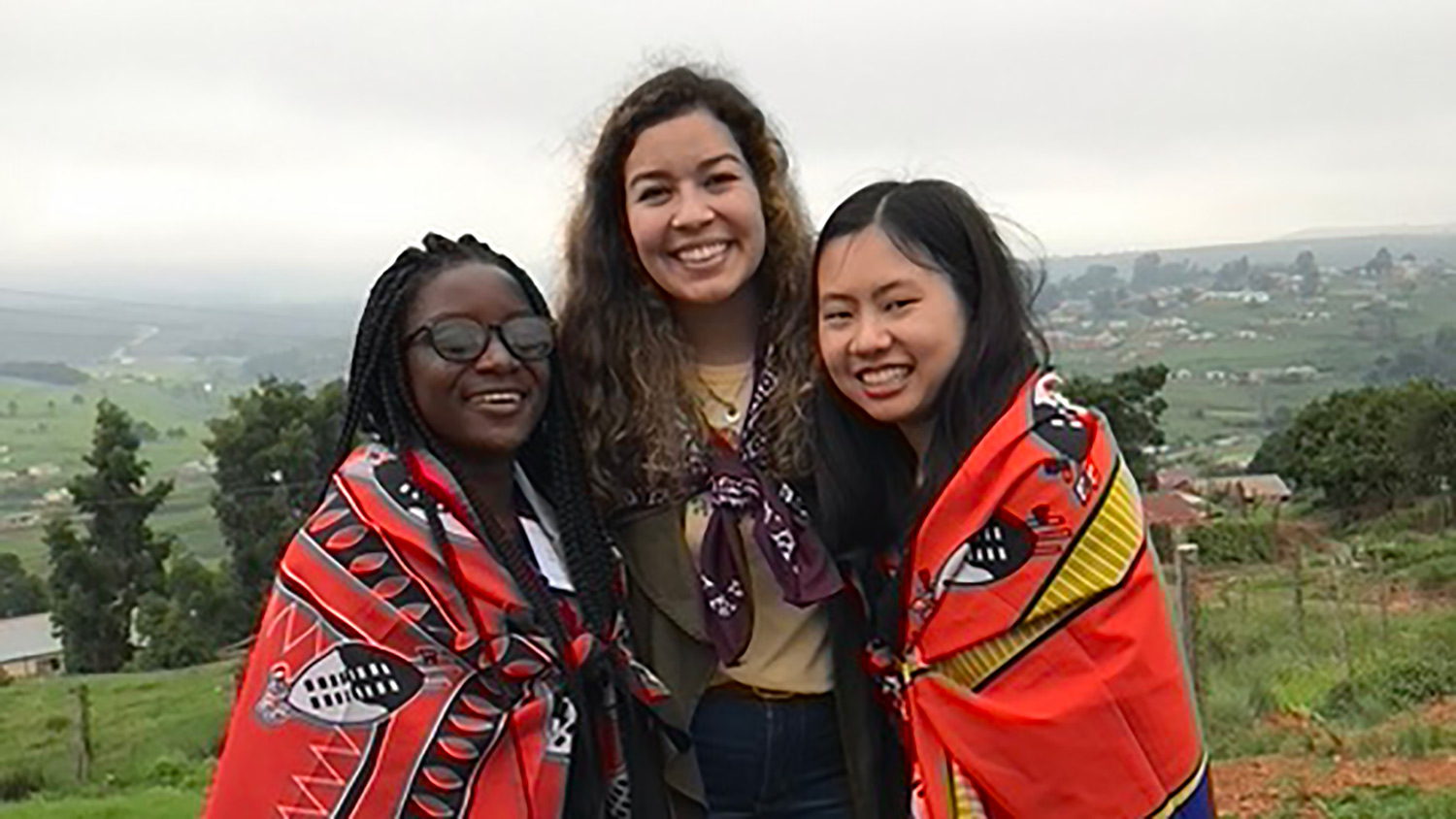
Junior Emily O’Rourke traveled to South Africa to investigate epidemics and returned with a broader outlook on her fundamental disease research.
Raleigh McElvery
March 31, 2020
Growing up in El Paso, Texas near the border of the U.S. and Mexico, Emily O’Rourke could venture across cultures in less time than it takes most people to commute to work. In fact, her dad would make this short trip each day for his job as a mechanical engineer. Watching him cross over so frequently reminded O’Rourke that “ideas and skills don’t stop at the border.” O’Rourke herself would visit Mexico to see relatives, and these experiences seeded aspirations to spearhead international scientific collaborations. Now a junior in Course 7 (Biology), O’Rourke is continuing to add stamps to her passport while exploring the global implications of disease research.
O’Rourke chose MIT because it offered a particularly wide array of study abroad programs, in addition to having top-tier research opportunities. One such study abroad program, MIT International Science and Technology Initiatives (MISTI), operates 25 regional programs, matching undergraduate and graduate students with fully-funded internship, research, and teaching opportunities in over 40 countries. The summer after her first year, O’Rourke participated in MISTI’s MIT-Italy Program in order to gain some research experience in the realm of urban planning. For six weeks, she investigated the urban effects of sea level rise while living in Venice.
When she returned to campus for her sophomore year, O’Rourke was intending to double major in physics and biology. But she ultimately opted to drop physics and pursue the life sciences once she started working in Becky Lamason’s lab in the Department of Biology.
“I started to see how biology worked on a practical level,” she says. “I get to experience a hands-on connection by running DNA on a gel and doing other experiments. During our weekly lab meetings, I witness scientific stories as they unfold.”
More recently, the duo has begun to examine how Sca4 may coopt another protein in the host cell, known as clathrin, for its own malicious means. “Sca4 is a really big protein and we still don’t know its entire structure,” O’Rourke says, “and we’re hoping to uncover some new functions.”The Lamason lab investigates how parasites hijack host cells processes in order to spread infection. O’Rourke is working with graduate student Cassandra Vondrak to probe the proteins that allow the tick-borne Rickettsia parkeri to migrate from one cell to the next. Their protein of interest, surface cell antigen 4 (Sca4), is secreted by the bacterium and binds to the host’s cell membrane, reducing the tension across the membrane and allowing Rickettsia to punch through to the neighboring cell. O’Rourke and Vondrak aim to determine how Rickettsia releases Sca4, in the hopes of piecing together a general mechanism by which pathogens propagate.
While O’Rourke was studying infectious disease on a cellular level, she heard about an opportunity to explore epidemics on a global scale. Each January, the Harvard-MIT Program in Health Sciences and Technology sponsors a two-week class in South Africa called Evolution of an Epidemic. The class, taught by Professor of the Practice Bruce Walker, covers the medical, scientific, and political responses to new diseases, focusing on the HIV/AIDS epidemic. Walker, who is also the director of the Ragon Institute of MGH, MIT and Harvard, is a world leader in the study of immune control and evasion in HIV infection. Since then, he’s developed strong connections and research partnerships in South Africa where the disease is most prevalent.
O’Rourke enrolled in Evolution of an Epidemic, and MISTI helped her to plan her trip. On January 16, she landed in Johannesburg, the first of three destinations. The cohort of students from MIT, Harvard, and the African Leadership Academy attended lectures, spoke with patients, and met medical professionals.
After Johannesburg, the class traveled to Durban where they visited traditional healers who were learning to administer HIV/AIDS tests as part of the iTeach program.
“We had the chance to ask these healers how they felt about interacting with Western medicine, and whether it clashed with their traditional values,” O’Rourke says. “They said HIV was so new that they couldn’t draw upon ancient wisdom from their ancestors to treat it. They were directing patients towards Western treatments because they’d seen the devastation the disease could cause.”
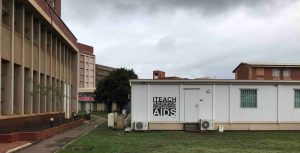
At their third and final destination, the province of KwaZulu-Natal, O’Rourke toured the FRESH Program. Twice a week, as part of a clinical trial, healthy African women around O’Rourke’s age attend classes that address topics like self-esteem, gender-based violence, HIV prevention, career development, and computer training. Before each session, the women are tested for HIV/AIDS, so if they contract it the researchers can treat it early and learn more about the disease’s initial stages.
“I really liked going there because it helped me see a direct connection between science and social good,” O’Rourke says. “It showed the value of talking to patients and asking about their experiences, rather than just looking at study outcomes.”
After two weeks, O’Rourke returned to MIT Biology and the Lamason lab with a broader outlook on her parasite research. “I’m able to see how my works fits into a larger context,” she says, “and how it may eventually have far-reaching impacts on disease evolution and spread.”
O’Rourke still plans to pursue fundamental biological research, but intends to seek out international collaborations focused on global health as well. It’s hard to leave the MIT bubble, she says, but it’s worth it. “Traveling can really broaden your perspective as a scientist, and inform your research in unexpected ways.”