Research Area: Stem Cell and Developmental Biology
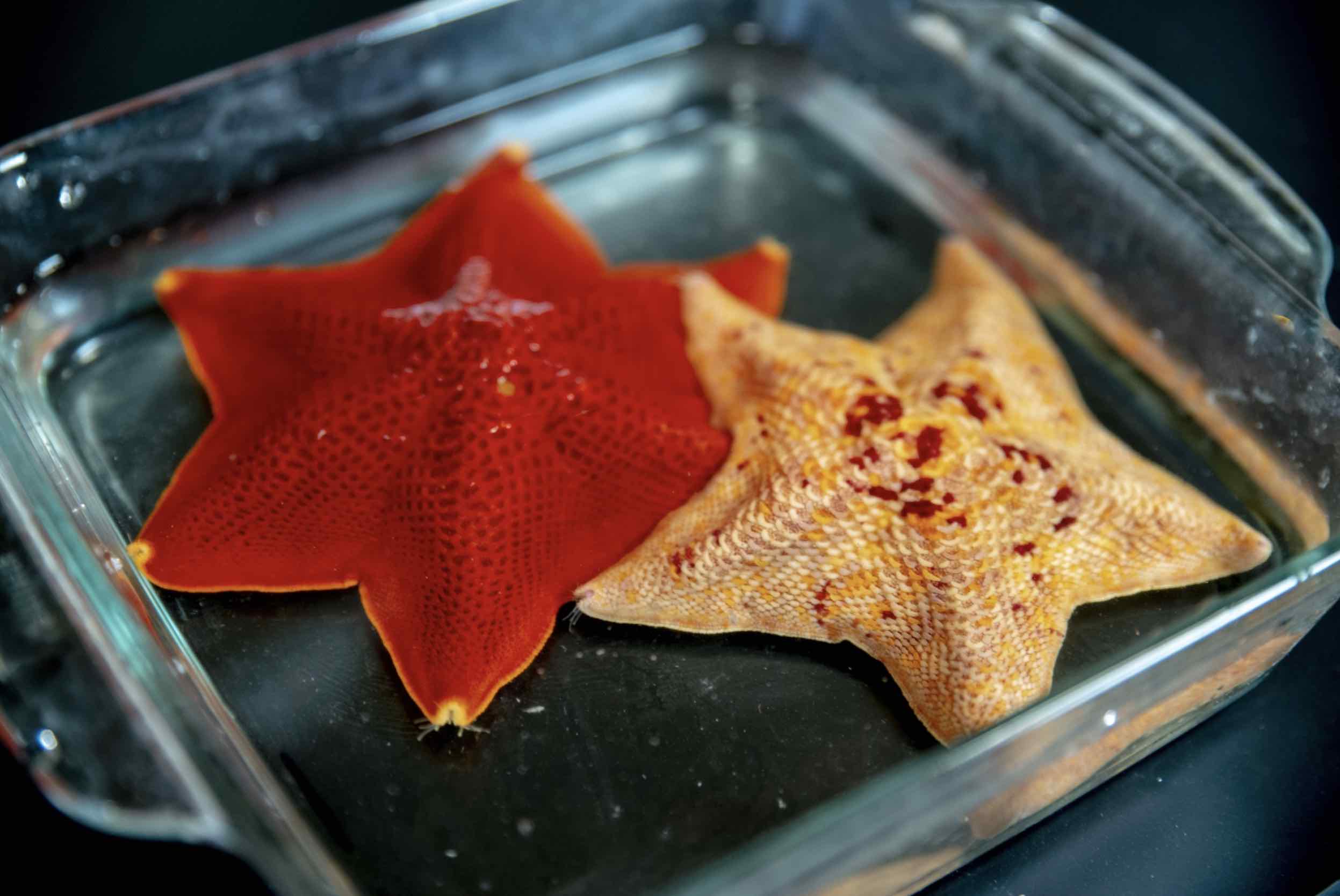
Greta Friar | Whitehead Institute
November 4, 2021
In a paper published Nov. 4 in the journal Current Biology, Zak Swartz, a postdoctoral researcher at Whitehead Institute, along with researchers in the lab of Whitehead Institute Member Iain Cheeseman and collaborators at the Massachusetts Institute of Technology (MIT), the University of Miami, and the Marine Biological Laboratory Embryology Course delve into the origins of the initial polarity in an animal’s first cell, which establishes an axis of symmetry for the developing organism and underlies the first steps of development. Their research reveals how a specific protein, called Dishevelled, localizes in a cell to help create this polarity.
All multicellular organisms begin as a single cell — the oocyte, precursor cell to the egg — which carries within it a “plan” for the fully developed, complex creature it will become. “How that multifunctional body plan is created is one of the deepest questions in developmental biology,” said Swartz.
“Sea stars, and a huge diversity of other animals, have an incredibly complex body plan, none of which is possible without the polarity of the initial cell,” said Cheeseman. “This work shows how the polarity originates as early as the meiotic divisions in the developing oocyte through an unexpected strategy to break its symmetry and achieve the asymmetric distribution of developmental factors.”
To study the intricate process of body patterning, Cheeseman Lab researchers used a type of sea star called the bat star, or Patiria miniata. These colorful animals are radially symmetric as adults — they usually have five arms, sometimes more — but as larvae they are bilaterally symmetric like humans.
The sea star larvae’s mirror-image symmetry is established when they are egg cells, called oocytes. A key step in the development of this organization involves a protein called Dishevelled, which localizes to the vegetal, or “bottom” end of the oocyte (which will define the posterior end of the embryo) as the cell gets ready to divide into two daughter cells.
Dishevelled — so named because a mutation in the homologous protein in fruit flies lends their tiny hairs a messy, tousled look — is a component of a common signaling pathway called the Wnt pathway, which is found in many creatures throughout the animal kingdom. The pathway serves various purposes in the cells, from body patterning to cell proliferation. “The Wnt pathway is evolutionarily ancient,” Swartz said. “Jellyfish use it, sea stars use it, people use it, and I think that’s really quite profound.”
In the sea stars, the pathway provides a link between the initial asymmetry of the oocyte and the polarity of the resulting embryo. Dishevelled serves as a messenger on the inside of the sea star’s cells, relaying external signals that are then transmitted through a molecular pathway to the cells’ nuclei.
The researchers used time-lapse imaging to visualize how Dishevelled moved around the oocyte as the cell went through different phases of its development. When the sea star oocyte was in a non-dividing phase, Dishevelled could be found distributed uniformly in small aggregations throughout the cytoplasm.
As the oocyte got ready to divide, however, Dishevelled aggregations dissolved and then reformed at the bottom of the cell at the furthest point from the nucleus. This provided a clear difference between the two ends of the oocyte.
Swartz was curious about how exactly the protein was localizing to the bottom of the oocyte. There were a number of protein transport options to investigate, so he began systematically ruling them out; the protein was not transported by the cell’s cytoskeleton (“You can think of these like little railroad tracks,” Swartz said), nor was it buoyed along on cytoplasmic currents, nor repelled by some factor at the “top” of the oocyte.
At this interval, Swartz reached out to two collaborators in MIT’s physics department, who helped design experiments to further probe the behavior of Dishevelled in the oocytes. “That’s when we started to consider the idea of dissolution and reassembly, which is kind of the punchline of the paper,” Swartz said. “You can think of it like salt crystals dissolving in water — rather than taking a pre-assembled thing and physically transporting it down [to the bottom of the oocyte], the idea is that these Dishevelled assemblies start out everywhere, get dissolved into their individual components, and then selectively reform in the vegetal region.”
The exact mechanism of this dissolution and reformation is not yet clear. Swartz was able to show that the reformation could not take place in the absence of another Wnt pathway protein called Frizzled, but because Frizzled is not exclusive to the bottom of the oocyte, it is not the only thing driving the reassembly.
In the future, Swartz plans to investigate whether the Dishevelled aggregates are formed in precise structures, or whether they group together as phase-separated droplets such as the RNA molecules studied in Whitehead Institute Member Ankur Jain’s lab or the protein molecules involved in transcription from Whitehead Institute Member Richard Young’s lab. “I’m interested in the broader composition of these structures,” he added. “Do they only contain Dishevelled, or are there other ingredients?”
Regardless of how the assemblies form, the new information on how Dishevelled localizes shines a light on a previously mysterious step in how the Wnt pathway plays a role in early body patterning in sea stars.
“It’s quite striking that Dishevelled localization seems to be an important feature in the Wnt pathway in sea stars, but also in distantly related vertebrates,” Swartz said. “My feeling is that the ability to activate this pathway in selective parts of the early embryo by interpreting polarity built into the oocyte may be a really critical feature of the evolution of the animal body plan.”
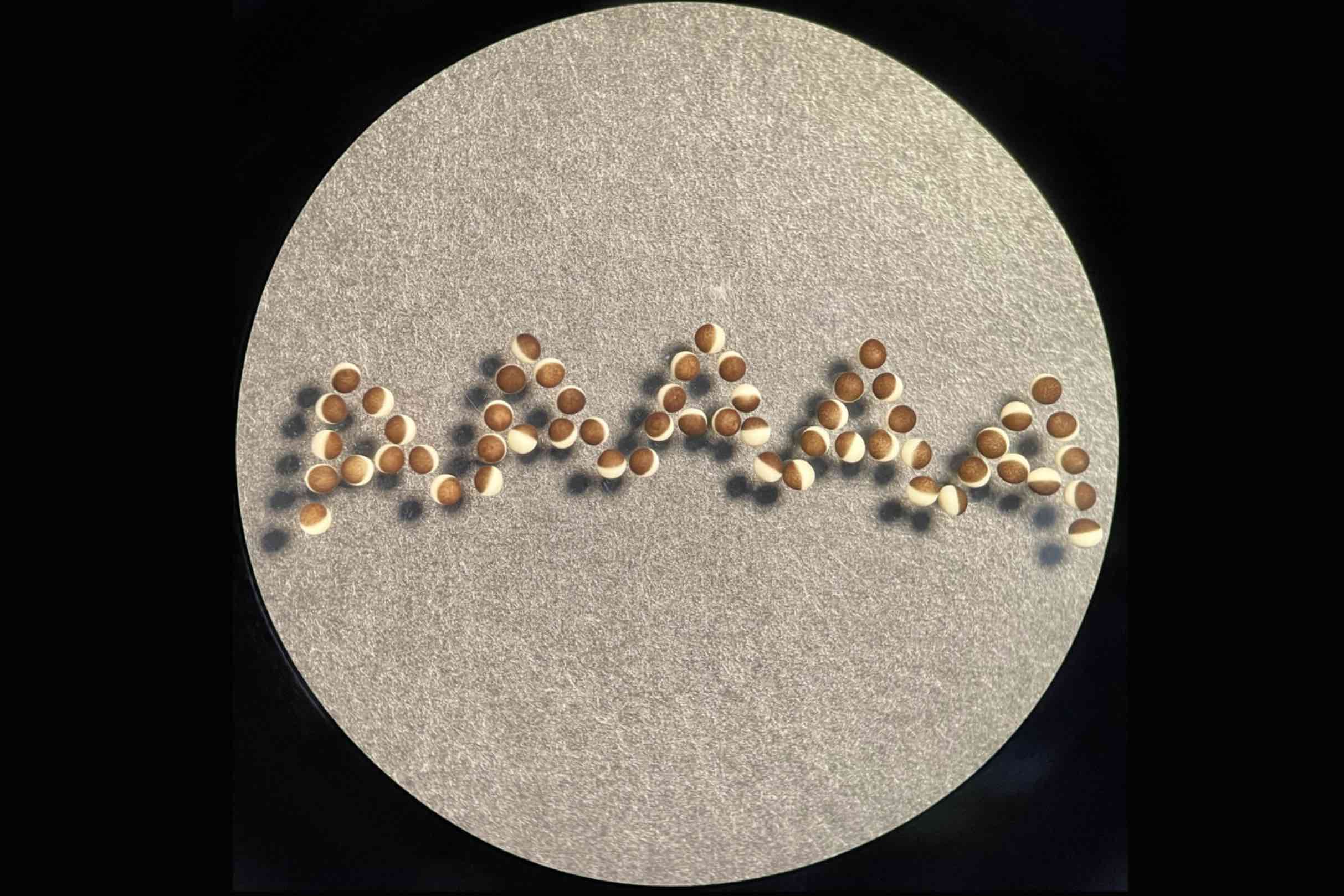
Greta Friar | Whitehead Institute
July 12, 2021
In new research, published in eLife on July 2, Bartel, who is also a professor of biology at Massachusetts Institute of Technology and an investigator with the Howard Hughes Medical Institute, and Bartel lab member Kehui Xiang, a CRI Irvington Postoctoral Fellow, have now discovered how cells establish this early gene regulatory regime and what conditions prompt a switch as the embryos mature. The researchers have observed the same regulatory switch in fish, frogs, and flies, and because the switch occurs across the animal kingdom, they would expect to see that the mechanism applies in other species including mammals.
“When I joined the lab, they had discovered that egg cells and early embryos had this different regulatory regime, and I wanted to know why,” Xiang says. “There must be fundamental changes to the cell, or to the molecules in the cell, that define this.”
The difference in how mRNAs are regulated during and after early development has to do with the length of their tails. mRNAs have tails made up of strings of adenines, one of the building blocks of RNA. Tail length varies between mRNAs from different genes and even between mRNAs from the same gene. Usually, the length of this “poly(A)-tail” corresponds to how long an mRNA lasts before getting degraded. An mRNA with a long tail is more stable, and will generally last longer. However, researchers had also observed that in some cases mRNA tail length corresponds to how readily an mRNA is used to make protein. Bartel’s earlier research had helped define when each of these connections occurs: mRNA tail length affects translational efficiency only in immature eggs and early embryos, and in other stages, it affects mRNA stability or lifespan.
In their new research, Xiang and Bartel uncovered three conditions that are required for the mRNA regulatory regime that exists in early development.
A competitive environment
The first condition is that there has to be a limited availability of a protein that binds to mRNA tails called cytoplasmic poly(A)-binding protein (PABPC). PABPC is known to help activate the translation of mRNA into protein. It binds to the mRNA tail and—in embryos—helps to increase translational efficiency; the researchers propose that it may do this by promoting a more favorable structure for translation. When PABPC is in limited supply, as it is in early embryos, then short-tailed mRNAs are less likely to bind any of the protein, as they will be outcompeted by long-tailed mRNAs, explaining the correlation between tail length and translational efficiency. Later in development, PABPC is in such ready supply that all of the mRNAs are able to bind at least one, decreasing the competitive edge of long-tailed mRNAs.
Early durability
However, the researchers observed that reducing the amount of PABPC in adult cells so that it becomes limiting in these cells did not cause mRNAs with longer tails to be translated more efficiently, which showed that other conditions must also contribute to early embryos’ unique regulation. The second condition that Xiang identified is that mRNAs must be relatively stable in spite of their inability to compete for PABPC. In adult cells, RNAs without PABPC bound to their tails are very unstable, and so are likely to degrade. If the same were true in early embryos, then the short-tailed mRNAs would degrade quickly because they are outcompeted for binding PABPC, and so one would again see a link between tail length and stability, rather than between tail length and translational efficiency—short-tailed mRNAs would be eliminated rather than poorly translated. However, the processes that would normally degrade mRNAs without PABPC have not yet started occurring in early embryos, allowing the short-tailed mRNAs to survive.
Big fish in a small pond
Finally, Xiang discovered that in order for tail length and translational efficiency to be linked, PABPC has to be able to affect translational efficiency. He found that in adult cells PABPC does not appear to boost translational efficiency the way it does in embryos. The researchers hypothesize that this is because the process of translating mRNAs in adult cells is already so efficient that the small boost from binding PABPC does not make a significant difference. However, in early embryos PABPC is more of a big fish in a small pond. The cells do not have all of the machinery to maximize translational efficiency, so every bit of improvement, such as the benefit of binding PABPC, makes a noticeable difference.
Together, these three conditions enable early eggs and embryos to regulate their mRNA in a unique fashion that can control how much protein is made from each gene without destroying the limited pool of mRNA available. In the future, the researchers hope to recreate the three conditions in non-embryonic cells to confirm that the conditions Xiang identified are not only necessary but also sufficient to cause the switch in regulatory regimes.
“Knowing which function the poly(A)-tail is performing in a specific cell or scenario—providing mRNA stability or translational efficiency—is really critical for understanding how genes are regulated in the different cells,” Bartel says. “And understanding that is important for answering all kinds of questions about cells, from their functions to what can go wrong with them in diseases.”
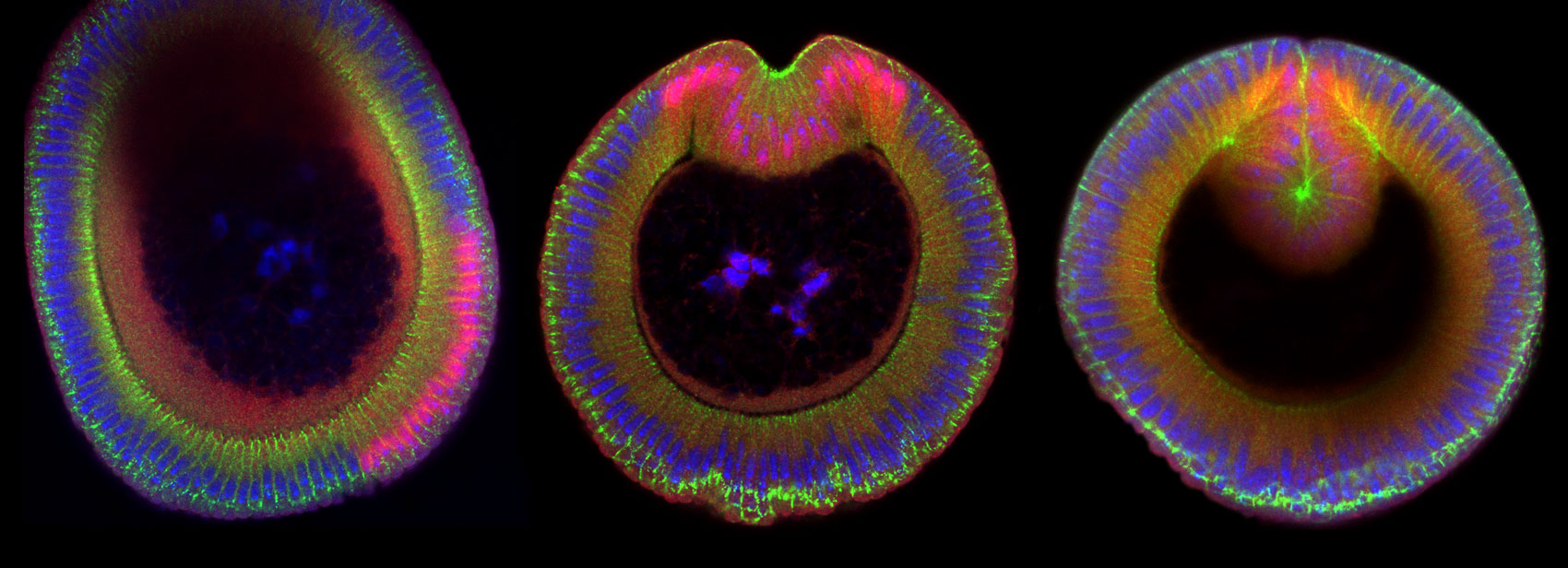
Patterns of myosin and F-actin proteins across developing embryos promote tissue folding and shape new life.
Raleigh McElvery
June 14, 2021
Virtually all multicellular organisms, including humans, begin as a single cell that rapidly divides and begets trillions of others. These cells work together, stretching, squishing, and migrating to sculpt organs and tissues. In the case of the fruit fly embryo, it only takes a few hours for life to take shape. First, the multiplying cells form an oblong sphere akin to a football. Then, mechanical forces cause a band of cells along the “belly” side of the developing fly to furrow inwards. These “mesoderm” cells form a new layer that will later give rise to muscles. Although the folding process transpires in less than 20 minutes, it’s crucial for determining where the cells will go and what roles they will assume.
Scientists previously identified two important proteins that generate the force needed to fold the tissue. The first, myosin, has a characteristic rod shape with feet hanging off both ends. It can “walk” along the cell’s inner scaffolding, composed of a second, rope-like protein called filamentous actin (F-actin). As it walks, myosin tugs on the F-actin and constricts the tissue. Researchers are probing the distribution of myosin and F-actin across the developing embryo, an important step towards understanding how these proteins drive constriction in the proper places to fold the tissue.
Myosin appears in a gradient across the belly and back of the developing fly. Since myosin and F-actin work together, many scientists assumed they would display the same pattern. However, new work from MIT’s Department of Biology and Department of Mathematics suggests otherwise. The study, published in the journal Development, shows how gene expression patterns dictate a unique distribution of F-actin across the mesoderm, which exhibits peaks and valleys. In combination with the myosin present, this F-actin pattern causes the cells to stretch, squish, or maintain their shape in just the right places to bend the tissue.
“We’ve known for decades that mechanical proteins like myosin and F-actin regulate tissue curvature during development,” says Adam Martin, an associate professor of biology and the study’s senior author. “But what hasn’t been appreciated is the extent to which these two proteins are intricately patterned during the tissue folding process. Our finding that F-actin has a different pattern than myosin was quite surprising.”
The researchers, led by graduate student Marlis Denk-Lobnig, began by focusing on two well-known transcription factor proteins, Twist and Snail, which bind to DNA to control gene expression. These transcription factors are known to dictate cell shape and fate during tissue folding, and Denk-Lobnig wondered how they affected F-actin levels.
By imaging live and fixed cells, the researchers observed that Snail and Twist drove a different pattern of F-actin density across the mesoderm compared to previously described myosin gradients. Two to three hours after the fruit fly eggs are laid, Snail depletes F-actin levels across the mesoderm. But, as Twist activates its transcriptional targets, F-actin and myosin levels rise in a subset of the mesoderm cells along the belly of the developing fly — constricting them and folding that swath of the tissue. The more F-actin and myosin a cell contains, the more compressed and wedge-shaped it becomes.
Denk-Lobnig also targeted another protein, RhoA, that tunes F-actin and myosin levels. RhoA activation is ultimately controlled by the ratio of two other molecules, C-GAP and RhoGEF2. The researchers adjusted the levels of C-GAP and RhoGEF2 in live cells, and watched the subsequent changes in myosin and F-actin distribution in real time.
To continue disentangling the effects of each protein on tissue curvature, they leveraged a computer simulation of a developing embryo designed by Associate Professor of Mathematics Jörn Dunkel, former grad student Pearson Miller PhD ’20, and postdoctoral researcher Jan Totz. The model allowed the team to adjust patterns of force and protein activity, in order to determine how the changes that they’d witnessed in real embryos affected tissue shape.
“The main takeaway is that you need this elegant coordination between cells during development,” Denk-Lobnig says. “We’ve shown how force generation patterns change the shape of individual cells — and how this leads to shape changes across entire tissues.”
Top image: Cross sections of three fruit fly embryos undergoing tissue folding. Nuclei are in blue, the transcription factor Snail is in red, and the junctional protein Armadillo is in green. Credit: Marlis Denk-Lobnig.
Video: An early stage fruit fly embryo has a band of cells on its surface that furrows inward to form a fold. Credit: Marlis Denk-Lobnig.
Citation:
“Combinatorial patterns of graded RhoA activation and uniform F-actin depletion promote tissue curvature”
Development, online June 14, DOI: 10.1242/dev.199232
Marlis Denk-Lobnig, Jan F. Totz, Natalie C. Heer, Jörn Dunkel, and Adam C. Martin
Posted: 6.14.21
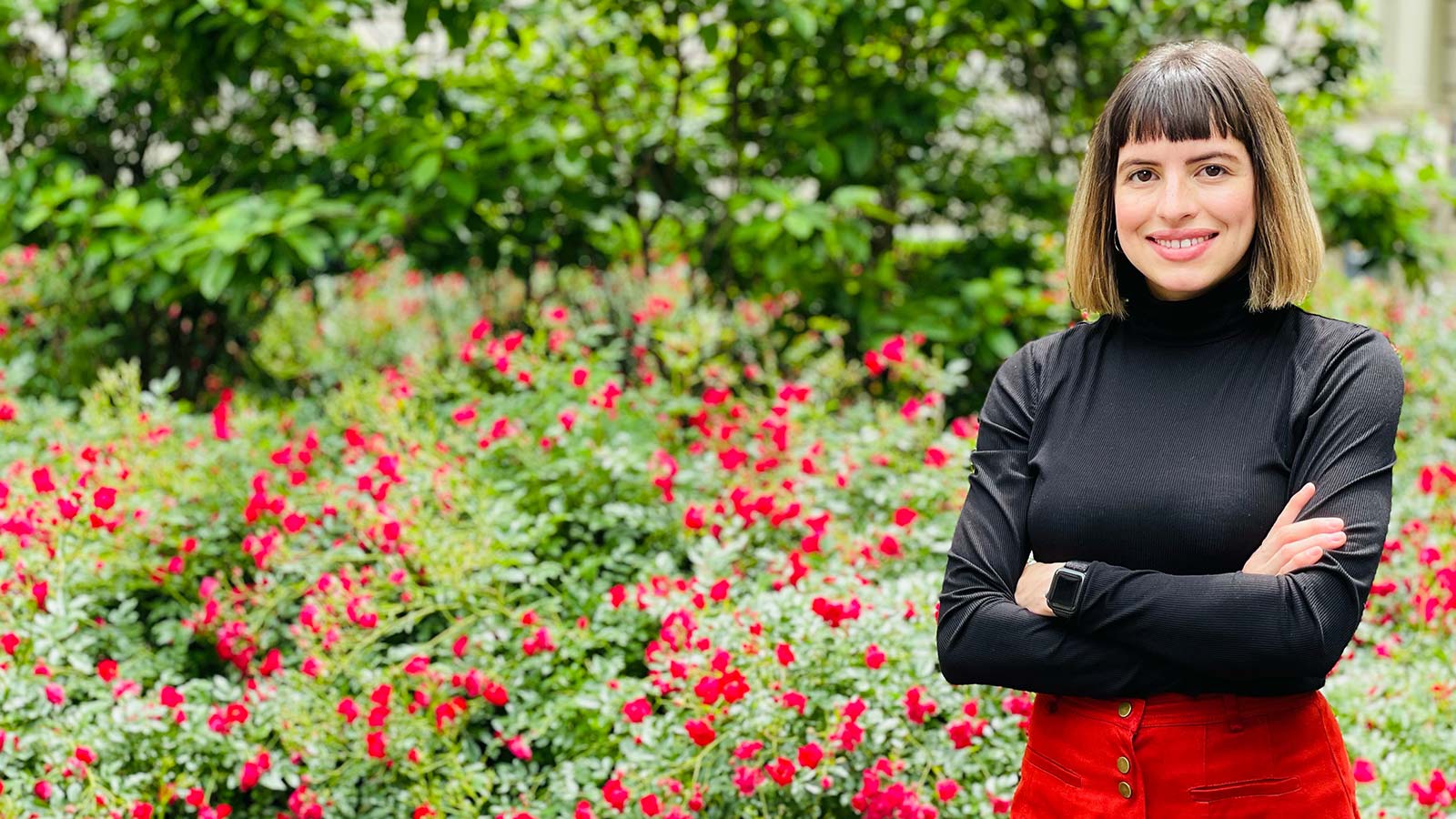
Education
- PhD, 2016, MIT
- BS, 2008, Chemistry, University of Puerto Rico-Mayagüez
Research Summary
We study chromatin — the complex of DNA and proteins that make up our chromosomes. We aim to understand how post-translational modifications to these building-blocks, as well as the factors that regulate these events, play essential roles in maintaining the integrity of cells, tissues, and ultimately entire organisms. We implement a combination of functional genomics, biochemical, genetic, and epigenomic approaches to study how chromatin and epigenetic factors decode the chemical language of chromatin, and how these are dysregulated in diseases such as cancer.
Awards
- AACR Gertrude B. Elion Cancer Research Award, 2023
- V Foundation Award, 2022
- NIH MOSAIC K99/R00 Postdoctoral Career Transition Award, 2021
- Eddie Méndez Scholar Award, Fred Hutchinson Cancer Research Center, 2020
-
Damon Runyon-Sohn Pediatric Cancer Fellowship, Damon Runyon Cancer Research Foundation, 2017
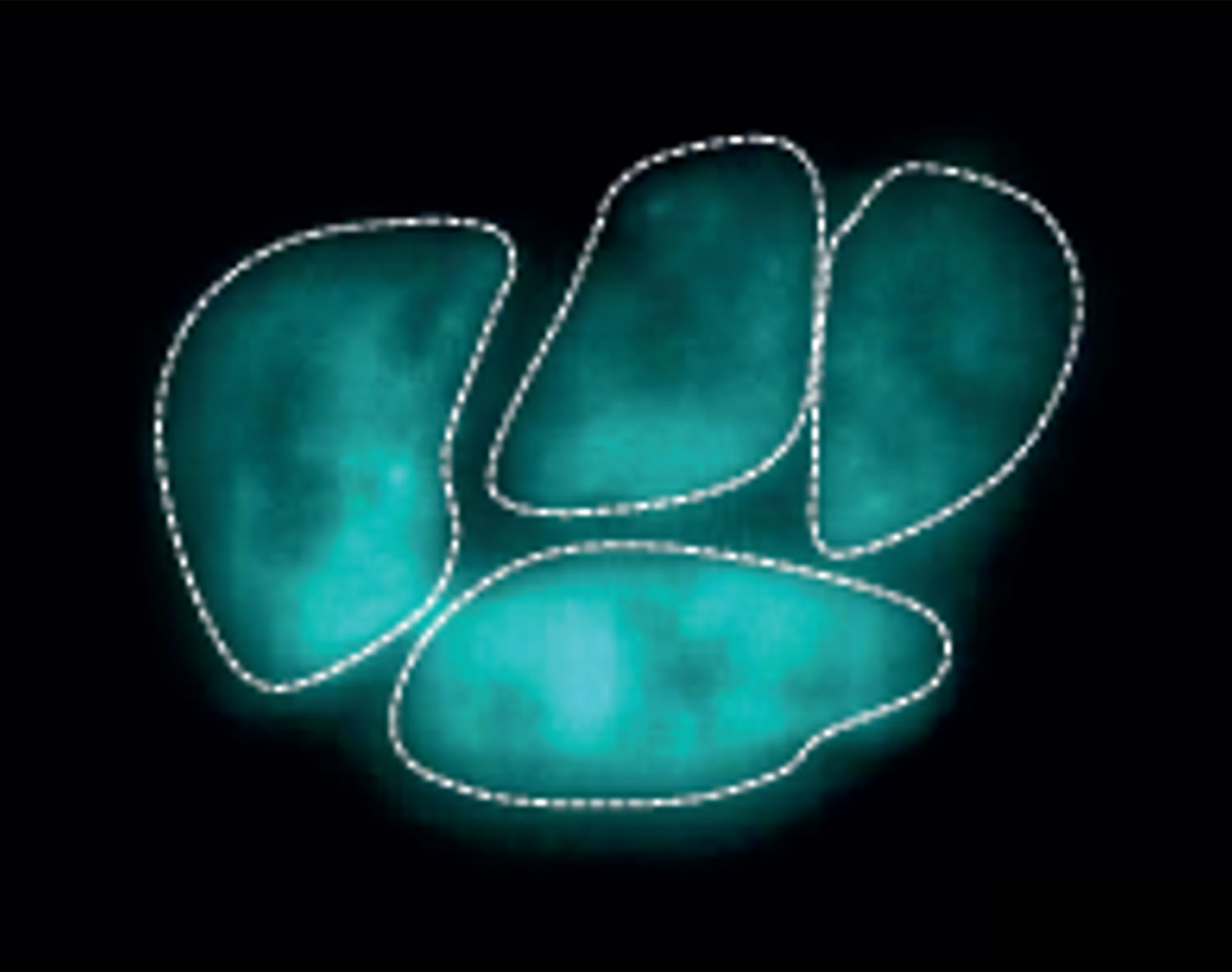
Raleigh McElvery
May 19, 2021
The DNA inside a single human cell is several meters long — yet it must be condensed to fit inside a space one-tenth the diameter of a hair. That’s like stretching a string from Philadelphia, Pennsylvania to Washington, D.C., and then trying to stuff it into a soccer ball. Imagine then organizing all of this information for each of the body’s 3 trillion cells! The DNA is condensed by proteins called histones that create a spool around which the DNA can wrap itself. How tightly the DNA is wound determines whether it is accessible enough for other proteins to bind to and copy into RNA, toggling gene expression levels up or down.
One specialized type of histone, H2A.Z, is ubiquitous and essential among multicellular organisms. But there have been conflicting reports about how it affects gene expression, especially during embryonic development.
Several years ago, Laurie Boyer’s lab at MIT was the first to show that H2A.Z wraps the DNA located around the start sites of most genes, where the molecular machine RNA polymerase II (RNAPII) binds to copy the DNA into RNA. Boyer’s team demonstrated that removing H2A.Z prevented embryonic cells from turning on genes that are important for forming organs and tissues. But scientists still weren’t sure how H2A.Z exerted its effects.
Now, in a recent Nature Structural and Molecular Biology study, a team from the Boyer lab, led by former postdoc Constantine Mylonas, has revealed how H2A.Z regulates the ability of RNAPII to properly transcribe DNA into the messages that specify all cell types in the body. The researchers found that in embryonic stem cells, H2A.Z serves as a “yellow traffic light,” signaling RNAPII to slow the process of transcribing DNA into RNA. Although there are other proteins that also contribute to RNAPII pausing, H2A.Z establishes a second barrier to transcription that allows gene expression to be tuned in response to developmental signals.
“H2A.Z appears to regulate how fast RNAPII begins to transcribe DNA, and this allows the cell time to respond to important cues that ultimately direct a stem cell to become a brain or heart cell, for example,” says Boyer, a professor of biology and biological engineering. “This connection was a critical missing piece of the puzzle, and explains why H2A.Z is essential for development across all multicellular organisms.”
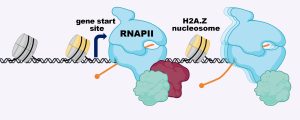
According to Boyer, H2A.Z’s role in gene expression has been difficult to pin down because previous approaches only provided static snapshots of how proteins interact with DNA days after loss of the histone. Boyer’s team overcame this shortcoming by leveraging a system that allowed for targeted degradation of H2A.Z within hours. They combined this technique with high-resolution genomic approaches and live cell imaging of RNAPII dynamics using super-resolution microscopy. With help from Ibrahim Cissé’s lab, they were able to visualize RNAPII dynamics in real time at the single molecule level in embryonic stem cells. Upon loss of H2A.Z, they found a remarkable increase in RNAPII movement in the cells, consistent with their genomic results showing a faster release of RNAPII and an increase in transcription in the absence of H2A.Z.
Next, the researchers plan to determine precisely how H2A.Z is targeted to the start sites of genes and how it forms a barrier to RNAPII passage.
Boyer says pinpointing the way histone variants like H2A.Z control gene expression is fundamental to understanding how developmental decisions are made, and will help researchers understand why misregulation of H2A.Z has been linked to diseases such as cancer.
“Emerging evidence indicates that DNA ‘packaging proteins’ like histones directly participate in how RNAPII can read and transcribe DNA,” she explains, “and that crucial connection wasn’t clear before.”
Image credits: courtesy of Laurie Boyer
Top image: Live cell super-resolution imaging showing RNAPII dynamics at a single molecule level in embryonic stem cells. The bright and colored clusters represent RNAPII molecules.
Citation:
“A dual role for H2A. Z. 1 in modulating the dynamics of RNA Polymerase II initiation and elongation.”
Nature Structural & Molecular Biology, online May 10, 2021, DOI: 10.1038/s41594-021-00589-3
Constantine Mylonas, Choongman Lee, Alexander L. Auld, Ibrahim I. Cisse, and Laurie A. Boyer.
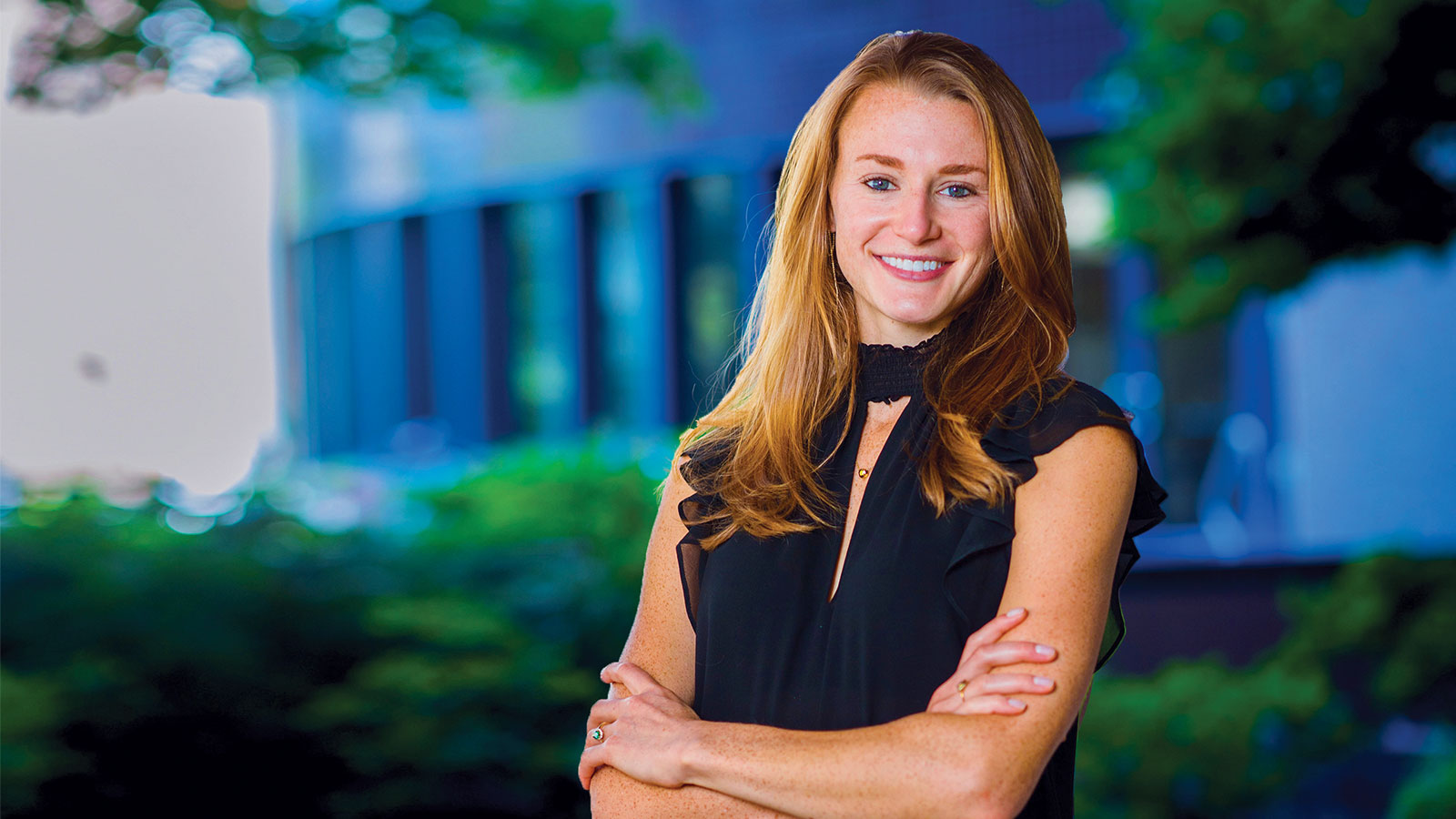
Education
- PhD, 2017, MIT; MD, 2018, Harvard Medical School
- Undergraduate: BS, 2010, Biology, Duke University
Research Summary
We aim to understand how tissues sense and respond to damage with the goal of developing novel treatments for diverse human diseases. We focus on the mammalian liver, which has the unique ability to completely regenerate itself, in order to identify the molecular requirements for effective organ repair. To this end, we innovate genetic, molecular, and cellular tools that allow us to investigate and modulate organ injury and regeneration directly within living organisms.
Awards
- NIH Director’s Early Independence Award, 2018
- Henry Asbury Christian Award, 2018
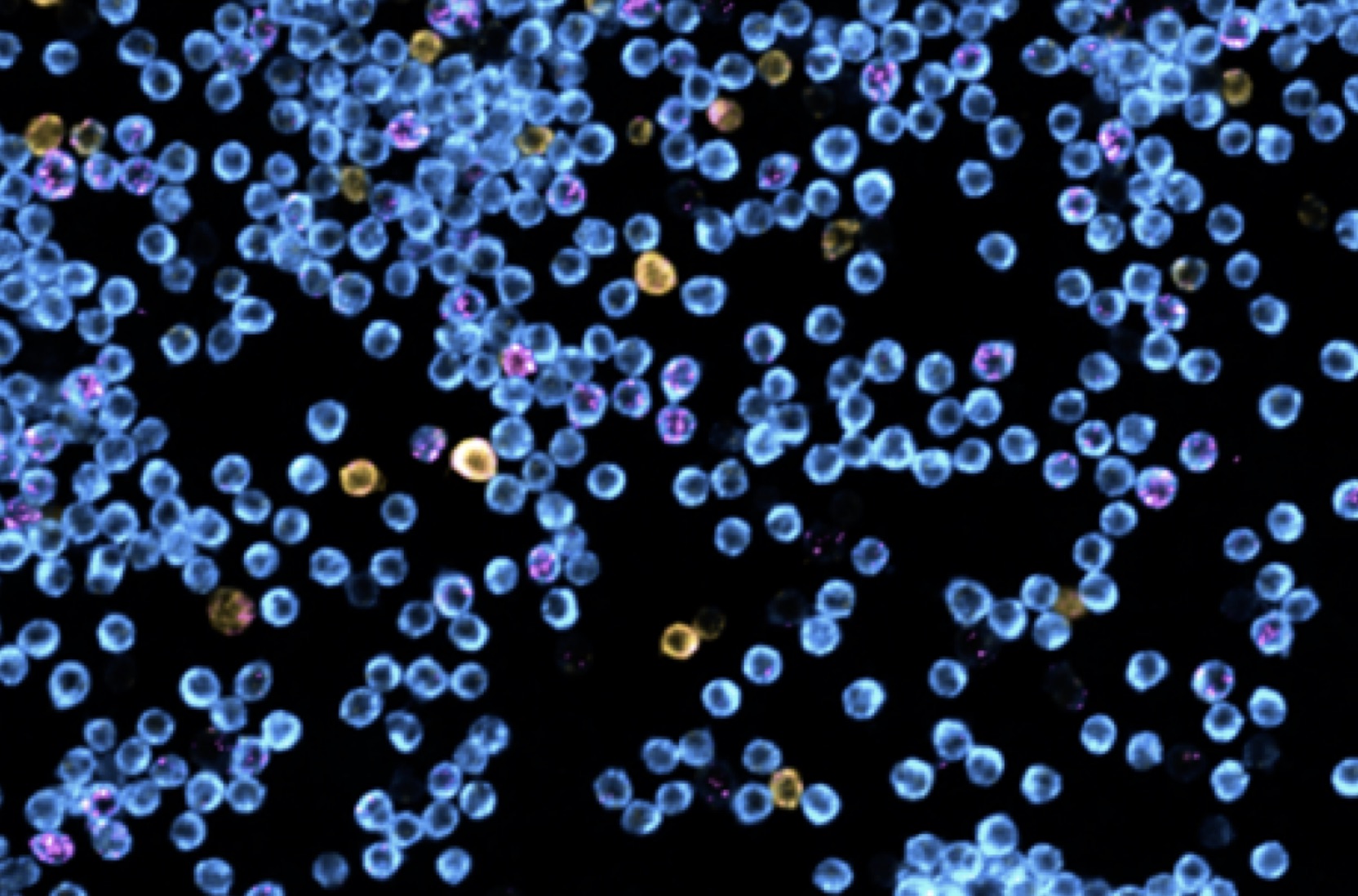
Eva Frederick | Whitehead Institute
April 20, 2021
Planarians are small water-dwelling worms known for their regenerative capacity. If you chop one into ten pieces, you’ll end up with ten fully-formed worms.
While humans have pools of specialized stem cells that can create our regenerative body parts like hair and skin, these worms owe their regenerative superpowers to a special kind of stem cell called a neoblast. At least some of these cells are “pluripotent,” meaning that they can divide to create almost any cell type in a worm’s body at any time. Neoblasts are actually the only dividing cells in planarians — fully committed cells like those in the eyes or intestines cannot divide again.
“The big question for us is, how does a neoblast go from being able to make anything, to making one particular thing?” says Amelie Raz, a postdoctoral researcher at Whitehead Institute who conducted her graduate research in the lab of Whitehead Institute Member Peter Reddien. “How do they go from being able to make anything in the body to being, say, an intestine cell that’s going to stay an intestine cell until it dies?”
Now, in a paper published online April 20 in the journal Cell Stem Cell, researchers at Whitehead Institute lay out a new model for how these stem cells commit to their fates and go on to create fully differentiated cells. The process of cellular differentiation is often viewed as a hierarchy, with one special stem cell at the top which can take a number of potential paths to arrive at a specialized state. This is generally thought to take place over a series of cell divisions in which each generation’s fate is gradually restricted.
“We’re proposing something happens that is very different from the conventional view,” says senior author Reddien, who is also a professor of biology at Massachusetts Institute of Technology and an investigator with the Howard Hughes Medical Institute. “We think that stem cells can make broad jumps in state without going through a series of fate-restricting divisions. We call it the single-step fate model.”
In the new model, neoblasts that are on a path toward creating skin cells or intestine cells can produce progeny cells that can switch fates to create cells of other types. The work is a step in the long road to understanding these worms’ regenerative capacities, and could possibly inform regenerative medicine approaches far in the future.
“The ability of planarian stem cells to essentially switch their fate is really, really powerful,” says Raz, the first author of the paper. “Obviously this is a long way off, but theoretically the concept of stem cell fate switching could be applied to regenerative medicine, with human stem cell programming.”
Upturning the hierarchy
Neoblasts can be sorted into many “classes.” For example, one class of neoblasts contains all the materials to make skin cells, and others have the necessary toolkit to form the worms’ primitive kidneys or their intestines. According to the hierarchical model, these specialized neoblasts are intermediaries between a pluripotent cell at the top of the hierarchy, and the non-dividing body cells.
“You can imagine that the special cell at the top is a blank slate with no predisposition towards any cell type — it can make anything,” says Raz. “This is how we’ve often imagined development works.”
But Raz, Reddien and Omri Wurtzel, a former postdoc in the Reddien lab now at Tel Aviv University, started to question this assumption after noticing a few mysterious properties of planarian cells.
First of all, researchers have observed in the past that when a planarian is treated with radiation to kill all existing stem cells, a single neoblast can rescue the animal by forming a colony containing many different classes of neoblasts. If, as previous theories suggested, there was a single class of neoblast that gave rise to all these types, Raz and Reddien reasoned that that class should be a common resident in every colony that formed. After creating many of these colonies and analyzing their composition, however, the researchers saw that this was not the case. “For every class we looked at, there were plenty of colonies that lacked that class altogether,” says Reddien. “There was no unique class present in all colonies.”
Another sticking point: the researchers began to realize that, when applying the hierarchy model, the math of planarian cell divisions and potency just didn’t add up. In a prior cell transplantation study, the Reddien lab found that many of the neoblasts they tested were pluripotent —in this study they found that proportion to be larger than what they would expect if only non-specialized neoblasts were pluripotent. “When you add up all the different kinds of specialized neoblasts, it’s at least three quarters of the neoblast population, and almost certainly higher than that.” says Raz. Therefore, the researchers wondered if some specialized neoblasts could be pluripotent as well.
Another study from the Reddien lab showed that skin-specialized neoblasts did not retain skin fate through more than one cell division. Also, in about half of all cell divisions in planarians, the two daughter cells will be different from one another. This raised the possibility that specialized neoblasts can divide asymmetrically as a possible route to stem cells changing fate.
Furthermore, the timeline for regeneration was off — the rate at which planarians were able to regrow body parts didn’t allow for several rounds of fate-restricting divisions.
After conducting experiments to study these different situations, Raz, Wurtzel, and Reddien were able to create a case for their new model of cell differentiation. “What we think is happening is that planarians have a ton of plasticity in their general stem cell population, where individual cells can move in and out of different specialized stages through the process of cell division in order to give rise to what is required,” Raz says.
“This is just the beginning of exploring this process, even though we’ve been studying it for many years,” Reddien says. “Focusing on the model, we’re suggesting that the cells can choose one fate, and then through the process of a division with an asymmetric outcome, one of the daughter cells can now divide again and choose a different fate. That fate switching process might be fundamental to explaining pluripotency.”
Reddien’s lab will continue investigating the mechanisms of neoblast fate specification, including how specialization lines up with the timing of the cell cycle.
“Understanding the structure of cell lineage and how fate choices are made is fundamental to understanding adult stem cell biology, and how in the context of injury and repair, new cells can be brought about,” says Reddien. “Do they have to go through long, complex lineage trajectories? Or can they make big jumps in state from stem cells to the final state? How flexible is that? All of these things have potential implications for understanding stem cell biology broadly, and we hope that the work will highlight some of these mechanisms and provide opportunities to explore general principles in the future.”
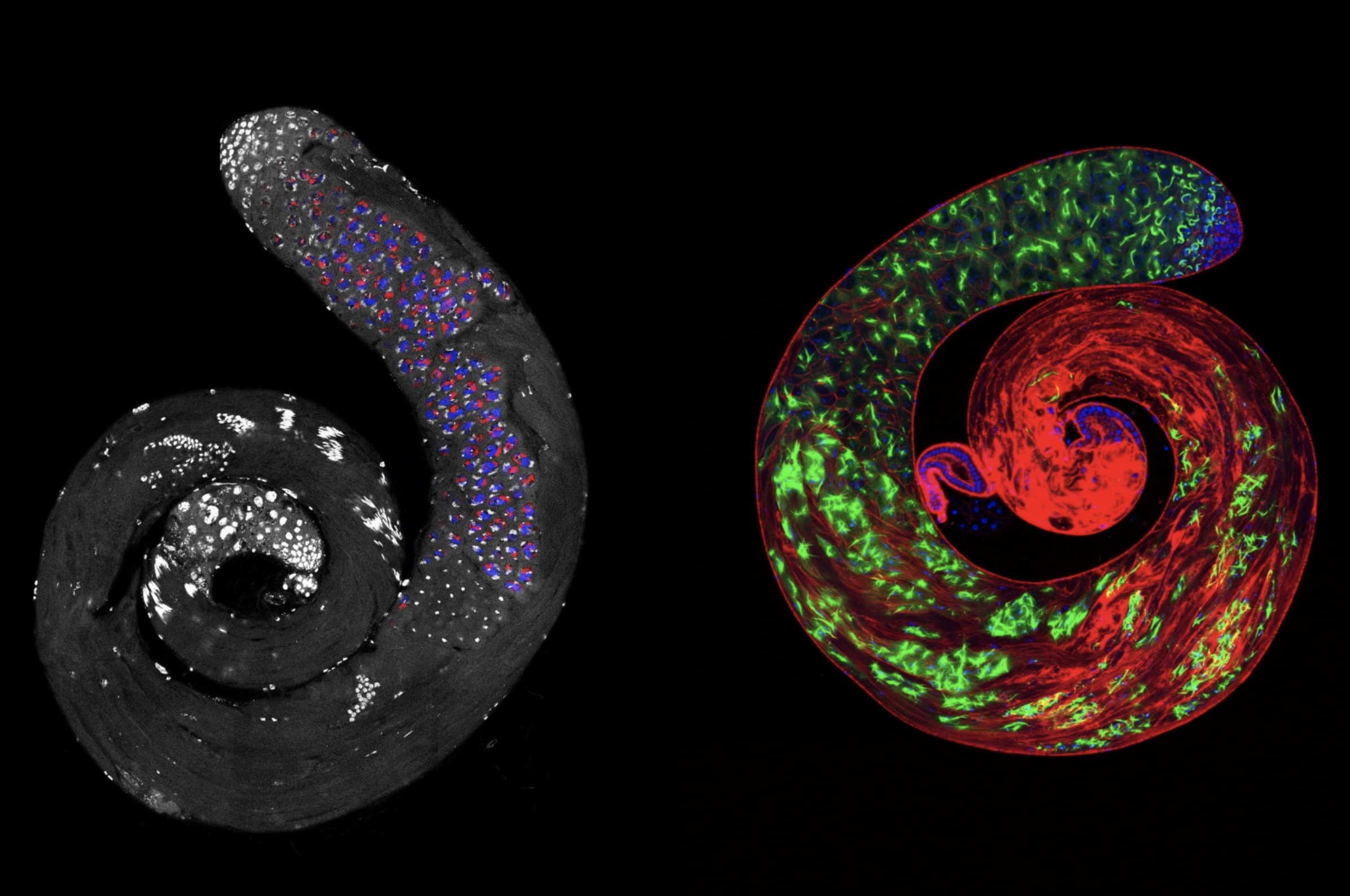
Eva Frederick | Whitehead Institute
March 16, 2021
New Whitehead Institute director Ruth Lehmann and new Member Yukiko Yamashita study opposite sides of the germ cell life cycle. Yamashita’s work in male germ cells shows how the cells are formed and maintained; Lehmann studies female germ cells to understand their fates. At the Whitehead Institute, they join Member and former director David Page in painting a fuller picture of how these seemingly immortal cell lines pass instructions uninterrupted from generation to generation.
All other cells in the body — neurons, muscle cells, the stem cells that replenish other tissue types — are made anew in each embryo and go away when organisms die. But not the germ cells. “The germ cell passes its DNA to the next generation, then that DNA is used to build up to a new germ cell,” says Yamashita. “That means that germ cells never cease to exist.”
In this way, an unbroken chain of germ cells stretches back to our most distant ancestor. Scientists study this never-ending link for insights into the fundamentals of biology and evolution. Yamashita began studying germ cells as a model to investigate other questions, but as her research progressed, she grew more and more intrigued by the cells’ special properties.
“This is one thing Ruth and I have in common,” Yamashita says. “There are many biologists that study germ cells, but not many are acutely interested or fascinated by this immortality. We want to know, where does it come from?”
Yamashita, also an Investigator of the Howard Hughes Medical Institute, joined Whitehead Institute in September. Work in her laboratory at Whitehead Institute will focus on two areas, using the fruit fly Drosophila melanogaster as a model. First, she will continue her focus from previous projects on the mechanics of asymmetric cell division using male germline stem cells. These cells, like other stem cells in the body, must undergo a series of asymmetric divisions — instead of simply dividing into two identical daughter cells, the cells must create daughters with different cell fates and programming.
“This balance — maintaining the stem cell number while making some differentiating cells — is considered to be a very important process,” she says. “If you end up making too many stem cells, it can become cancerous; but if you commit too much to the differentiation, you lose the stem cell count, and that means you cannot continue sperm production.”
A newer project in her lab centers on the long sequences of nucleotides within organisms’ genomes that don’t code for any genes. They’re often nonsensical, gibberish combinations or long strings of certain bases. This “genomic junk” has long been dismissed as meaningless filler between essential genes, but Yamashita proposes that the junk is essential for the overall structure of the genome. Much like the binding of a book holds together its contents in an organized fashion, the genomic junk may provide a blueprint for how genetic material is held together and eventually read.
Ultimately, it is the germline cells that are responsible for maintaining this DNA framework. Yamashita hypothesizes that slow changes in junk DNA could provide some explanation for why different species are reproductively incompatible.
“If you look at the chimpanzee genome and the human genome, the protein coding regions are, like, 98 percent, 99 percent identical,” she says. “But the junk DNA part is very, very different. We think this divergence might explain what happens when one species splits into two.”
Yamashita’s research team will share lab space with Lehmann’s group. Both researchers use fruit flies for their experiments, but Lehmann’s research focuses on egg cells, not sperm. “Germ cells are special; you don’t need them for survival, but you need them to keep the species going,” she says. “How are they initially specified and set aside? What makes them different, how are they set aside from somatic cells, and how do they maintain their cell fate?”
One project Lehmann is carrying over from her work at New York University’s Skirball Institute of Biomolecular Medicine involves phase transition condensates — small, membraneless granules that bring together the components needed for complex cellular functions. Lehmann studies a specific type of condensate called a germ granule, an aggregation of small RNAs and RNA binding proteins found only in germline cells, which helps determine the cells’ fate.
Lehmann is also investigating the female germline cells’ role in maternal inheritance. After fertilization, the maternal cell imparts not only its nuclear DNA but also components of its cytoplasm, including mitochondria, RNAs, and even bacteria. “This whole idea of cytoplasmic inheritance and the transgenerational continuum of the cytoplasm is something I’m just starting to think about,” she says.
Yamashita and Lehmann share a large open space on the third floor of the Institute, with researchers from each lab integrated throughout. They will also share a fly room and computational room. The researchers hope the communal setup will allow a flow of ideas between their labs. “By sharing this kind of basic space, we are hoping to let our people interact with each other and for discussions to happen,” Yamashita says.
“This is a new concept for Whitehead, and we’ll see how it works,” Lehmann says. “It’s an exciting experiment in lab sociology.”
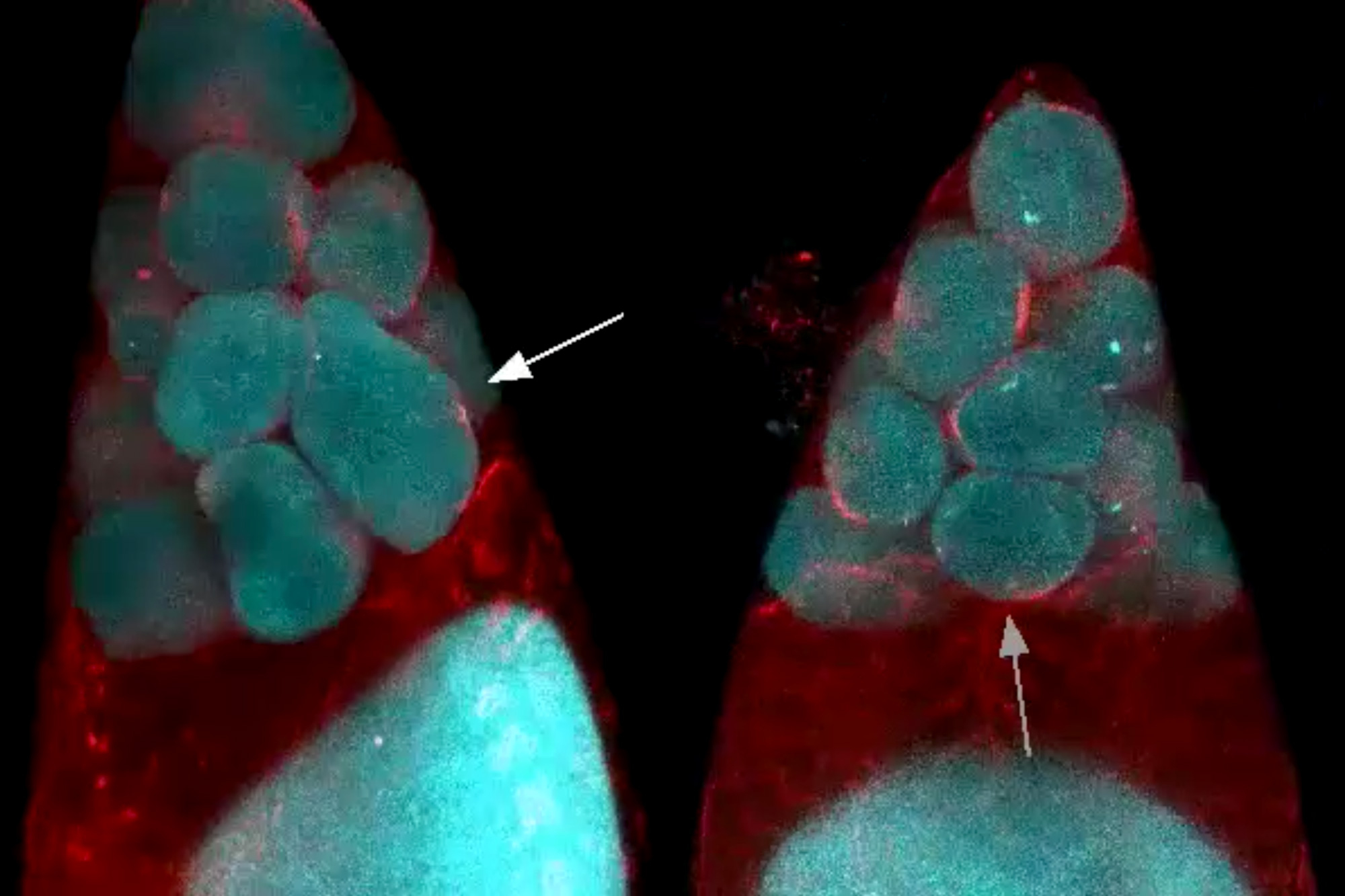
Oocyte growth relies on physical phenomena that drive smaller cells to dump their contents into a larger cell.
Anne Trafton | MIT News Office
March 10, 2021
Egg cells are by far the largest cells produced by most organisms. In humans, they are several times larger than a typical body cell and about 10,000 times larger than sperm cells.
There’s a reason why egg cells, or oocytes, are so big: They need to accumulate enough nutrients to support a growing embryo after fertilization, plus mitochondria to power all of that growth. However, biologists don’t yet understand the full picture of how egg cells become so large.
A new study in fruit flies, by a team of MIT biologists and mathematicians, reveals that the process through which the oocyte grows significantly and rapidly before fertilization relies on physical phenomena analogous to the exchange of gases between balloons of different sizes. Specifically, the researchers showed that “nurse cells” surrounding the much larger oocyte dump their contents into the larger cell, just as air flows from a smaller balloon into a larger one when they are connected by small tubes in an experimental setup.
“The study shows how physics and biology come together, and how nature can use physical processes to create this robust mechanism,” says Jörn Dunkel, an MIT associate professor of physical applied mathematics. “If you want to develop as an embryo, one of the goals is to make things very reproducible, and physics provides a very robust way of achieving certain transport processes.”
Dunkel and Adam Martin, an MIT associate professor of biology, are the senior authors of the paper, which appears this week in the Proceedings of the National Academy of Sciences. The study’s lead authors are postdoc Jasmin Imran Alsous and graduate student Nicolas Romeo. Jonathan Jackson, a Harvard University graduate student, and Frank Mason, a research assistant professor at Vanderbilt University School of Medicine, are also authors of the paper.
A physical process
In female fruit flies, eggs develop within cell clusters known as cysts. An immature oocyte undergoes four cycles of cell division to produce one egg cell and 15 nurse cells. However, the cell separation is incomplete, and each cell remains connected to the others by narrow channels that act as valves that allow material to pass between cells.
Members of Martin’s lab began studying this process because of their longstanding interest in myosin, a class of proteins that can act as motors and help muscle cells contract. Imran Alsous performed high-resolution, live imaging of egg formation in fruit flies and found that myosin does indeed play a role, but only in the second phase of the transport process. During the earliest phase, the researchers were puzzled to see that the cells did not appear to be increasing their contractility at all, suggesting that a mechanism other than “squeezing” was initiating the transport.
“The two phases are strikingly obvious,” Martin says. “After we saw this, we were mystified, because there’s really not a change in myosin associated with the onset of this process, which is what we were expecting to see.”
Martin and his lab then joined forces with Dunkel, who studies the physics of soft surfaces and flowing matter. Dunkel and Romeo wondered if the cells might be behaving the same way that balloons of different sizes behave when they are connected. While one might expect that the larger balloon would leak air to the smaller until they are the same size, what actually happens is that air flows from the smaller to the larger.
This happens because the smaller balloon, which has greater curvature, experiences more surface tension, and therefore higher pressure, than the larger balloon. Air is therefore forced out of the smaller balloon and into the larger one. “It’s counterintuitive, but it’s a very robust process,” Dunkel says.
Adapting mathematical equations that had already been derived to explain this “two-balloon effect,” the researchers came up with a model that describes how cell contents are transferred from the 15 small nurse cells to the large oocyte, based on their sizes and their connections to each other. The nurse cells in the layer closest to the oocyte transfer their contents first, followed by the cells in more distant layers.
“After I spent some time building a more complicated model to explain the 16-cell problem, we realized that the simulation of the simpler 16-balloon system looked very much like the 16-cell network. It is surprising to see that such counterintuitive but mathematically simple ideas describe the process so well,” Romeo says.
The first phase of nurse cell dumping appears to coincide with when the channels connecting the cells become large enough for cytoplasm to move through them. Once the nurse cells shrink to about 25 percent of their original size, leaving them only slightly larger than their nuclei, the second phase of the process is triggered and myosin contractions force the remaining contents of the nurse cells into the egg cell.
“In the first part of the process, there’s very little squeezing going on, and the cells just shrink uniformly. Then this second process kicks in toward the end where you start to get more active squeezing, or peristalsis-like deformations of the cell, that complete the dumping process,” Martin says.
Cell cooperation
The findings demonstrate how cells can coordinate their behavior, using both biological and physical mechanisms, to bring about tissue-level behavior, Imran Alsous says.
“Here, you have several nurse cells whose job it is to nurse the future egg cell, and to do so, these cells appear to transport their contents in a coordinated and directional manner to the oocyte,” she says.
Oocyte and early embryonic development in fruit flies and other invertebrates bears some similarities to those of mammals, but it’s unknown if the same mechanism of egg cell growth might be seen in humans or other mammals, the researchers say.
“There’s evidence in mice that the oocyte develops as a cyst with other interconnected cells, and that there is some transport between them, but we don’t know if the mechanisms that we’re seeing here operate in mammals,” Martin says.
The researchers are now studying what triggers the second, myosin-powered phase of the dumping process to start. They are also investigating how changes to the original sizes of the nurse cells might affect egg formation.
The research was funded by the National Institute of General Medical Sciences, a Complex Systems Scholar Award from the James S. McDonnell Foundation, and the Robert E. Collins Distinguished Scholarship Fund.