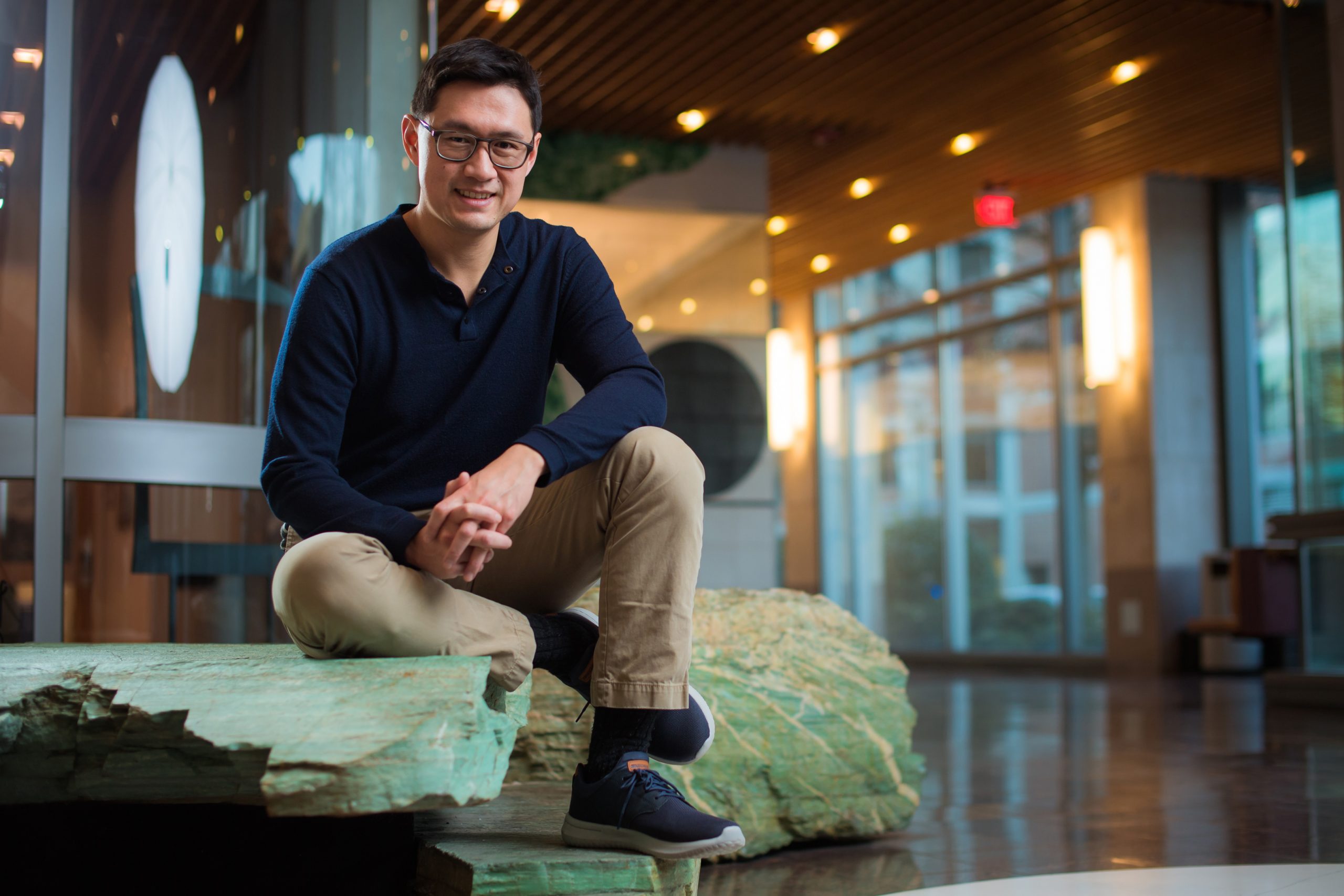
Gene-Wei Li investigates the rules that cells use to maintain the correct ratio of the proteins they need to survive.
Anne Trafton | MIT News Office
January 4, 2023
A typical bacterial genome contains more than 4,000 genes, which encode all of the proteins that the cells need to survive. How do cells know just how much of each protein they need for their everyday functions?
Gene-Wei Li, an MIT associate professor of biology, is trying to answer that question. A physicist by training, he uses genome-wide measurements and biophysical modeling to quantify cells’ protein production and discover how cells achieve such precise control of those quantities.
Using those techniques, Li has found that cells appear to strictly control the ratios of proteins that they produce, and that these ratios are consistent across cell types and across species.
“Coming from a physics background, it’s surprising to me that these cells have evolved to be really precise in making the right amount of their proteins,” Li says. “That observation was enabled by the fact that we are able to design measurements with a precision that matches what is actually happening in biology.”
From physics to biology
Li’s parents — his father, a marine biologist who teaches at a university in Taiwan, and his mother, a plant biologist who now runs a science camp for high school students — passed their affinity for science on to Li, who was born in San Diego while his parents were graduate students there.
The family returned to Taiwan when Li was 2 years old, and Li soon became interested in math and physics. In Taiwan, students choose their college major while still in high school, so he decided to study physics at National Tsinghua University.
While in college, Li was drawn to optical physics and spectroscopy. He went to Harvard University for graduate school, where after his first year, he started working in a lab that works on single-molecule imaging of biological systems.
“I realized there are a lot of really exciting fields at the boundary between disciplines. It’s something that we didn’t have in Taiwan, where the departments are very strict that physics is physics, and biology is biology,” Li says. “Biology is a lot messier than physics, and I had some hesitancy, but I was happy to see that biology does have rules that you can observe.”
For his PhD research, Li used single-molecule imaging to study proteins called transcription factors — specifically, how quickly they can bind to DNA and initiate the copying of DNA into RNA. Though he had never taken a class in biology, he began to learn more about it and decided to do a postdoc at the University of California at San Francisco, where he worked in the lab of Jonathan Weissman, a professor of cellular and molecular pharmacology.
Weissman, who is now a professor of biology at MIT, also trained as a physicist before turning to biology. In Weissman’s lab, Li developed techniques for studying gene expression in bacterial cells, using high-throughput DNA sequencing. In 2015, Li joined the faculty at MIT, where his lab began to work on tools that could be used to measure gene expression in cells.
When genes are expressed in cells, the DNA is first copied into RNA, which carries the genetic instructions to ribosomes, where proteins are assembled. Li’s lab has developed ways to measure protein synthesis rates in cells, along with the amount of RNA that is transcribed from different genes. Together, these tools can yield precise measurements of how much a particular gene is expressed in a given cell.
“We had the qualitative tools before, but now we can really have quantitative information and learn how much protein is made and how important those protein levels are to the cell,” Li says.
Precise control
Using these tools, Li and his students have discovered that different species of bacteria can have different strategies for making proteins. In E. coli, transcription of DNA and translation of RNA into proteins had long been known to be a coupled process, meaning that after RNA is produced, ribosomes immediately translate it into protein.
Many researchers assumed that this would be true for all bacteria, but in a 2020 study, Li found that Bacillus subtilis and hundreds of other bacterial species use a different strategy.
“A lot of other species have what we call runaway transcription, where the transcription happens really fast and the proteins don’t get made at the same time. And because of this uncoupling, these species have very different mechanisms of regulating their gene expression,” Li says.
Li’s lab has also found that across species, cells make the same proportions of certain proteins that work together. Many cellular processes, such as breaking down sugar and storing its energy as ATP, are coordinated by enzymes that perform a series of reactions in a specified sequence.
“Evolution, it turns out, gives us the same proportion of those enzymes, whether in E. coli or other bacteria or in eukaryotic cells,” Li says. “There are apparently rules and principles for designing these pathways that we didn’t know of before.”
Mutations that cause too much or too little of a protein to be produced can cause a variety of human diseases. Li now plans to investigate how the genome encodes the rules governing the correct quantities of each protein, by measuring how changes to genetic and regulatory sequences affect gene expression at each step of the process — from initiation of transcription to protein assembly.
“The next level that we’re trying to focus on is: How is that information stored in the genome?” he says. “You can easily read off protein sequences from a genome, but it’s still impossible to tell how much protein is going to be made. That’s the next chapter.”