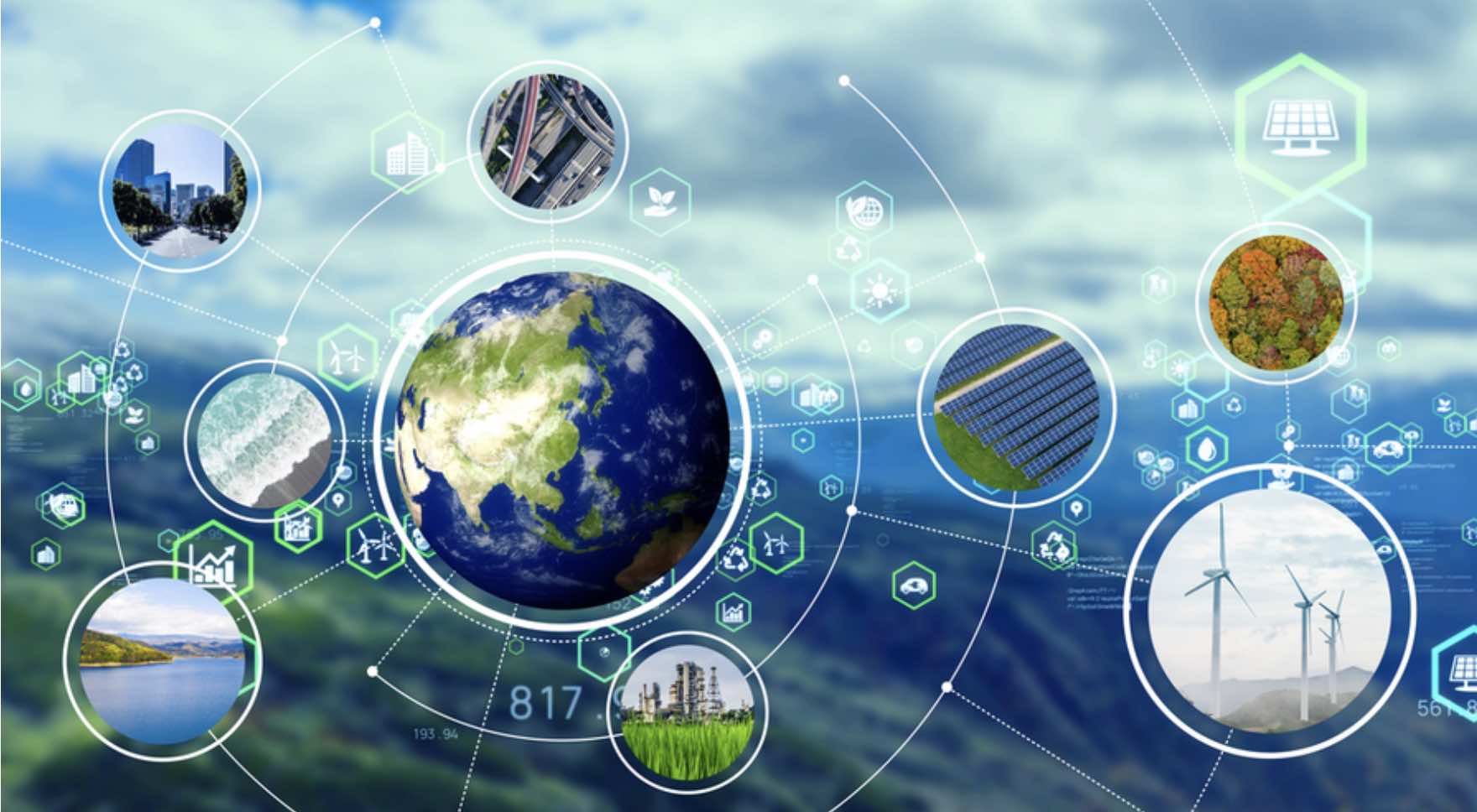
Twenty winning projects will link industry member priorities with research groups across campus to develop scalable climate solutions.
Molly Chase | Climate and Sustainability Consortium
May 23, 2022
The MIT Climate and Sustainability Consortium (MCSC) has awarded 20 projects a total of $5 million over two years in its first-ever 2022 MCSC Seed Awards program. The winning projects are led by principal investigators across all five of MIT’s schools.
The goal of the MCSC Seed Awards is to engage MIT researchers and link the economy-wide work of the consortium to ongoing and emerging climate and sustainability efforts across campus. The program offers further opportunity to build networks among the awarded projects to deepen the impact of each and ensure the total is greater than the sum of its parts.
For example, to drive progress under the awards category Circularity and Materials, the MCSC can facilitate connections between the technologists at MIT who are developing recovery approaches for metals, plastics, and fiber; the urban planners who are uncovering barriers to reuse; and the engineers, who will look for efficiency opportunities in reverse supply chains.
“The MCSC Seed Awards are designed to complement actions previously outlined in Fast Forward: MIT’s Climate Action Plan for the Decade and, more specifically, the Climate Grand Challenges,” says Anantha P. Chandrakasan, dean of the MIT School of Engineering, Vannevar Bush Professor of Electrical Engineering and Computer Science, and chair of the MIT Climate and Sustainability Consortium. “In collaboration with seed award recipients and MCSC industry members, we are eager to engage in interdisciplinary exploration and propel urgent advancements in climate and sustainability.”
By supporting MIT researchers with expertise in economics, infrastructure, community risk assessment, mobility, and alternative fuels, the MCSC will accelerate implementation of cross-disciplinary solutions in the awards category Decarbonized and Resilient Value Chains. Enhancing Natural Carbon Sinks and building connections to local communities will require associations across experts in ecosystem change, biodiversity, improved agricultural practice and engagement with farmers, all of which the consortium can begin to foster through the seed awards.
“Funding opportunities across campus has been a top priority since launching the MCSC,” says Jeremy Gregory, MCSC executive director. “It is our honor to support innovative teams of MIT researchers through the inaugural 2022 MCSC Seed Awards program.”
The winning projects are tightly aligned with the MCSC’s areas of focus, which were derived from a year of highly engaged collaborations with MCSC member companies. The projects apply across the member’s climate and sustainability goals.
The MCSC’s 16 member companies span many industries, and since early 2021, have met with members of the MIT community to define focused problem statements for industry-specific challenges, identify meaningful partnerships and collaborations, and develop clear and scalable priorities. Outcomes from these collaborations laid the foundation for the focus areas, which have shaped the work of the MCSC. Specifically, the MCSC Industry Advisory Board engaged with MIT on key strategic directions, and played a critical role in the MCSC’s series of interactive events. These included virtual workshops hosted last summer, each on a specific topic that allowed companies to work with MIT and each other to align key assumptions, identify blind spots in corporate goal-setting, and leverage synergies between members, across industries. The work continued in follow-up sessions and an annual symposium.
“We are excited to see how the seed award efforts will help our member companies reach or even exceed their ambitious climate targets, find new cross-sector links among each other, seek opportunities to lead, and ripple key lessons within their industry, while also deepening the Institute’s strong foundation in climate and sustainability research,” says Elsa Olivetti, the Esther and Harold E. Edgerton Associate Professor in Materials Science and Engineering and MCSC co-director.
As the seed projects take shape, the MCSC will provide ongoing opportunities for awardees to engage with the Industry Advisory Board and technical teams from the MCSC member companies to learn more about the potential for linking efforts to support and accelerate their climate and sustainability goals. Awardees will also have the chance to engage with other members of the MCSC community, including its interdisciplinary Faculty Steering Committee.
“One of our mantras in the MCSC is to ‘amplify and extend’ existing efforts across campus; we’re always looking for ways to connect the collaborative industry relationships we’re building and the work we’re doing with other efforts on campus,” notes Jeffrey Grossman, the Morton and Claire Goulder and Family Professor in Environmental Systems, head of the Department of Materials Science and Engineering, and MCSC co-director. “We feel the urgency as well as the potential, and we don’t want to miss opportunities to do more and go faster.”
The MCSC Seed Awards complement the Climate Grand Challenges, a new initiative to mobilize the entire MIT research community around developing the bold, interdisciplinary solutions needed to address difficult, unsolved climate problems. The 27 finalist teams addressed four broad research themes, which align with the MCSC’s focus areas. From these finalist teams, five flagship projects were announced in April 2022.
The parallels between MCSC’s focus areas and the Climate Grand Challenges themes underscore an important connection between the shared long-term research interests of industry and academia. The challenges that some of the world’s largest and most influential companies have identified are complementary to MIT’s ongoing research and innovation — highlighting the tremendous opportunity to develop breakthroughs and scalable solutions quickly and effectively. Special Presidential Envoy for Climate John Kerry underscored the importance of developing these scalable solutions, including critical new technology, during a conversation with MIT President L. Rafael Reif at MIT’s first Climate Grand Challenges showcase event last month.
Both the MCSC Seed Awards and the Climate Grand Challenges are part of MIT’s larger commitment and initiative to combat climate change. Underscored in “Fast Forward: MIT’s Climate Action Plan for the Decade,” which the Institute published in May 2021.
The project titles and research leads for each of the 20 awardees listed below are categorized by MCSC focus area.
Decarbonized and resilient value chains
- “Collaborative community mapping toolkit for resilience planning,” led by Miho Mazereeuw, associate professor of architecture and urbanism in the Department of Architecture and director of the Urban Risk Lab (a research lead on Climate Grand Challenges flagship project) and Nicholas de Monchaux, professor and department head in the Department of Architecture
- “CP4All: Fast and local climate projections with scientific machine learning — towards accessibility for all of humanity,” led by Chris Hill, principal research scientist in the Department of Earth, Atmospheric and Planetary Sciences and Dava Newman, director of the MIT Media Lab and the Apollo Program Professor in the Department of Aeronautics and Astronautics
- “Emissions reductions and productivity in U.S. manufacturing,” led by Mert Demirer, assistant professor of applied economics at the MIT Sloan School of Management and Jing Li, assistant professor and William Barton Rogers Career Development Chair of Energy Economics in the MIT Sloan School of Management
- “Logistics electrification through scalable and inter-operable charging infrastructure: operations, planning, and policy,” led by Alex Jacquillat, the 1942 Career Development Professor and assistant professor of operations research and statistics in the MIT Sloan School of Management
- “Powertrain and system design for LOHC-powered long-haul trucking,” led by William Green, the Hoyt Hottel Professor in Chemical Engineering in the Department of Chemical Engineering and postdoctoral officer, and Wai K. Cheng, professor in the Department of Mechanical Engineering and director of the Sloan Automotive Laboratory
- “Sustainable Separation and Purification of Biochemicals and Biofuels using Membranes,” led by John Lienhard, the Abdul Latif Jameel Professor of Water in the Department of Mechanical Engineering, director of the Abdul Latif Jameel Water and Food Systems Lab, and director of the Rohsenow Kendall Heat Transfer Laboratory; and Nicolas Hadjiconstantinou, professor in the Department of Mechanical Engineering, co-director of the Center for Computational Science and Engineering, associate director of the Center for Exascale Simulation of Materials in Extreme Environments, and graduate officer
- “Toolkit for assessing the vulnerability of industry infrastructure siting to climate change,” led by Michael Howland, assistant professor in the Department of Civil and Environmental Engineering
Circularity and Materials
- “Colorimetric Sulfidation for Aluminum Recycling,” led by Antoine Allanore, associate professor of metallurgy in the Department of Materials Science and Engineering
- “Double Loop Circularity in Materials Design Demonstrated on Polyurethanes,” led by Brad Olsen, the Alexander and I. Michael Kasser (1960) Professor and graduate admissions co-chair in the Department of Chemical Engineering, and Kristala Prather, the Arthur Dehon Little Professor and department executive officer in the Department of Chemical Engineering
- “Engineering of a microbial consortium to degrade and valorize plastic waste,” led by Otto Cordero, associate professor in the Department of Civil and Environmental Engineering, and Desiree Plata, the Gilbert W. Winslow (1937) Career Development Professor in Civil Engineering and associate professor in the Department of Civil and Environmental Engineering
- “Fruit-peel-inspired, biodegradable packaging platform with multifunctional barrier properties,” led by Kripa Varanasi, professor in the Department of Mechanical Engineering
- “High Throughput Screening of Sustainable Polyesters for Fibers,” led by Gregory Rutledge, the Lammot du Pont Professor in the Department of Chemical Engineering, and Brad Olsen, Alexander and I. Michael Kasser (1960) Professor and graduate admissions co-chair in the Department of Chemical Engineering
- “Short-term and long-term efficiency gains in reverse supply chains,” led by Yossi Sheffi, the Elisha Gray II Professor of Engineering Systems, professor in the Department of Civil and Environmental Engineering, and director of the Center for Transportation and Logistics
- The costs and benefits of circularity in building construction, led by Siqi Zheng, the STL Champion Professor of Urban and Real Estate Sustainability at the MIT Center for Real Estate and Department of Urban Studies and Planning, faculty director of the MIT Center for Real Estate, and faculty director for the MIT Sustainable Urbanization Lab; and Randolph Kirchain, principal research scientist and co-director of MIT Concrete Sustainability Hub
Natural carbon sinks
- “Carbon sequestration through sustainable practices by smallholder farmers,” led by Joann de Zegher, the Maurice F. Strong Career Development Professor and assistant professor of operations management in the MIT Sloan School of Management, and Karen Zheng the George M. Bunker Professor and associate professor of operations management in the MIT Sloan School of Management
- “Coatings to protect and enhance diverse microbes for improved soil health and crop yields,” led by Ariel Furst, the Raymond A. (1921) And Helen E. St. Laurent Career Development Professor of Chemical Engineering in the Department of Chemical Engineering, and Mary Gehring, associate professor of biology in the Department of Biology, core member of the Whitehead Institute for Biomedical Research, and graduate officer
- “ECO-LENS: Mainstreaming biodiversity data through AI,” led by John Fernández, professor of building technology in the Department of Architecture and director of MIT Environmental Solutions Initiative
- “Growing season length, productivity, and carbon balance of global ecosystems under climate change,” led by Charles Harvey, professor in the Department of Civil and Environmental Engineering, and César Terrer, assistant professor in the Department of Civil and Environmental Engineering
Social dimensions and adaptation
- “Anthro-engineering decarbonization at the million-person scale,” led by Manduhai Buyandelger, professor in the Anthropology Section, and Michael Short, the Class of ’42 Associate Professor of Nuclear Science and Engineering in the Department of Nuclear Science and Engineering
- “Sustainable solutions for climate change adaptation: weaving traditional ecological knowledge and STEAM,” led by Janelle Knox-Hayes, the Lister Brothers Associate Professor of Economic Geography and Planning and head of the Environmental Policy and Planning Group in the Department of Urban Studies and Planning, and Miho Mazereeuw, associate professor of architecture and urbanism in the Department of Architecture and director of the Urban Risk Lab (a research lead on a Climate Grand Challenges flagship project)