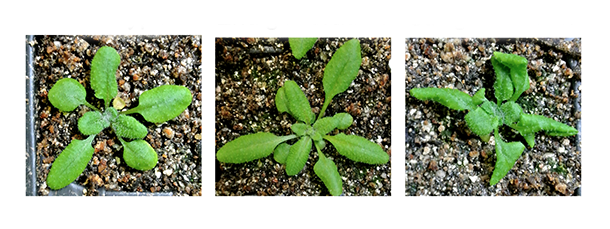
December 14, 2017
While our genome contains a vast repertoire of genes that are responsible for virtually all of the cellular and developmental processes life requires, it is the complex dance of regulating their expression that is vital for genetic programs to be executed successfully. Genes must be turned on and off at appropriate times or, in some cases, never turned on or off at all.
Methylation—the addition of chemical tags to DNA—typically reduces the expression of methylated genes. In many cases, DNA methylation can be thought of as roadblocks on a gene. The more methylated a gene is, the less likely it is that it will be active. Such genetic demarcations are critical to ensure that genes involved in particular stages of development are active at the right time, for example. Methylation is essential for proper cellular function, and its dysregulation is associated with diseases, such as cancer in humans. Despite its importance, little is known about how critical methylation patterns are inherited or maintained. Whitehead Institute Member Mary Gehring and her lab have identified a mechanism important for maintaining methylation, that when disrupted, results in the demethylation of large sections of the Arabidopsis plant’s genome. Their work is described this week in the journal Nature Communications.
Using an unusual gene in the plant Arabidopsis, Gehring is teasing apart the mechanisms that underpin methylation. By breaking this unique gene’s “circuit,” Gehring and Ben Williams, a postdoctoral researcher in her lab, have gained important insights into how methylation is maintained, including a surprising finding that previously erased methylation can be restored under certain circumstances.
In order to better understand methylation’s heritability, Gehring and Williams looked closely at an anomaly, the ROS1 gene in Arabidopsis plants, which encodes a protein that removes methylation from its own gene as well as others. Previously, Gehring and Williams had determined that ROS1 methylation actually functions in the complete opposite way from the existing paradigm—unlike most genes, when a short section of this gene is methylated, the gene is actually activated instead of inactivated. Conversely, if it is methylated, the gene is turned on. As a result, ROS1 can act as a rheostat for the Arabidopsis genome: As methylation increases, ROS1 turns on and begins removing methyl groups, and as methylation decreases, ROS1 shuts off and reduces its demethylating activity.
In the current research, Williams altered methylation at ROS1 so that its activity was uncoupled from methylation levels in the genome, in order to see what effects such a change would have on methylation throughout the entire genome. When he analyzed the plants’ methylation, it was haywire. Methylation was lost throughout the genome and progressively decreased in subsequent generations, except in a particular part of the genome called the heterochromatin—genomic areas that are strongly repressed. Interestingly, Williams found that, despite the alteration of the ROS1regulatory circuit, these heterochromatic sections of the genome actually regain their methylation and approach full methylation by the fourth generation— the same time point by which the rest of the genome has lost much of its methylation .
The researchers determined that the ROS1 circuit they uncovered is important for methylation homeostasis because it causes heritable loss of methylation when disrupted. And yet methylation returns at some locations, albeit not immediately, suggesting that Arabidopsis enlists multiple mechanisms to maintain methylation homeostasis. Gehring and Williams are intrigued by that delay in remethylation and are working to identify its cause as well as other mechanisms that may also be at work regulating this critical process.
This work was supported by the National Institute of General Medical Sciences of the National Institutes of Health (R01GM112851).