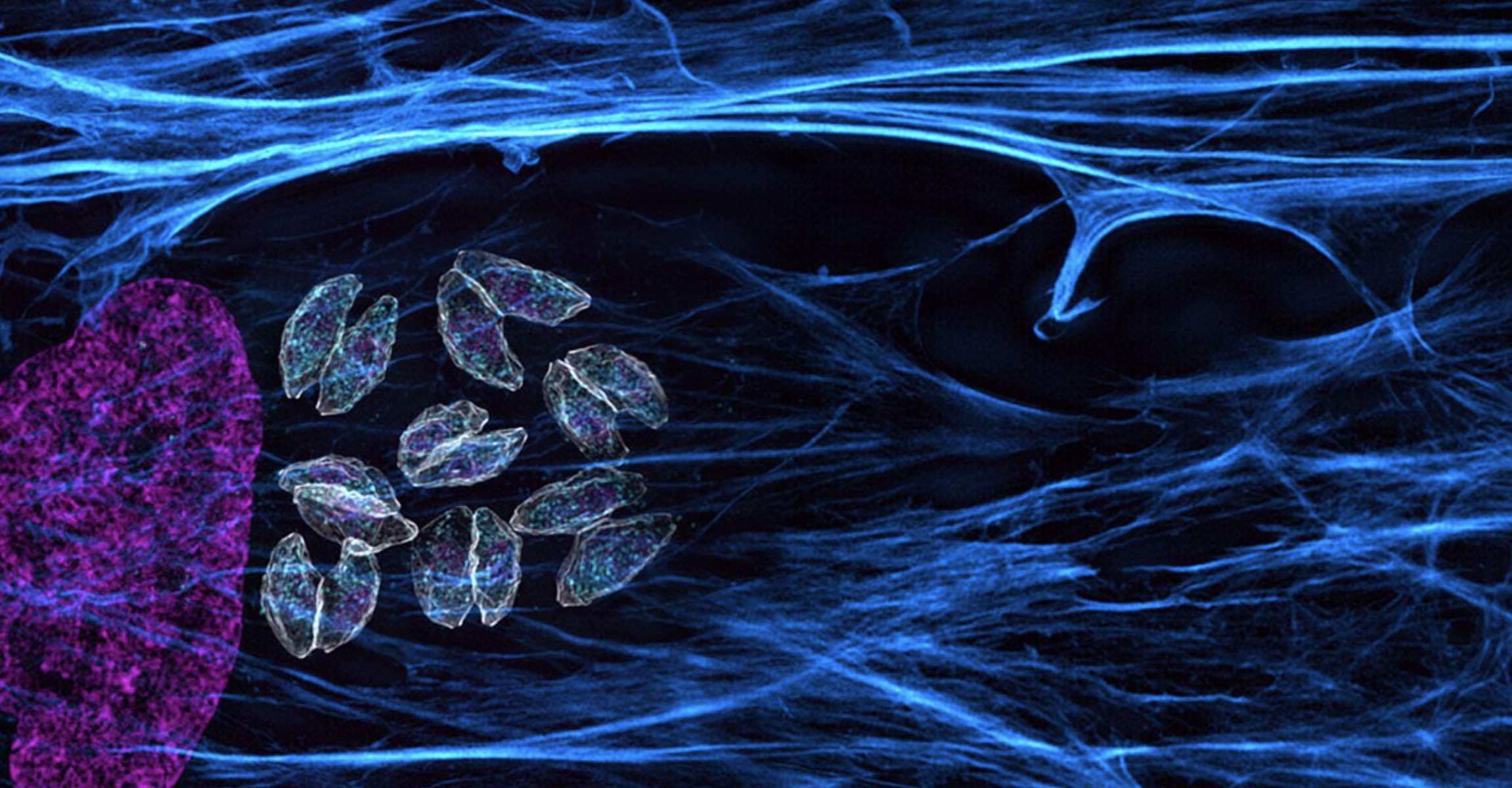
Eva Frederick | Whitehead Institute
September 23, 2020
The essential malaria drug artemisinin acts like a “ticking time bomb” in parasite cells — but in the half a century since the drug was introduced, malaria-causing parasites have slowly grown less and less susceptible to the treatment, threatening attempts at global control over the disease.
In a paper published September 23 in Nature Communications, Whitehead Institute Member Sebastian Lourido and colleagues use genome screening techniques in the related parasite Toxoplasma gondii (T. gondii) to identify genes that affect the parasites’ susceptibility to artemisinin. Two genes stood out in the screen: one that makes the drug more lethal, and another that helps the parasite survive the treatment.
Artemisinin is derived from the extract of sweet wormwood (Artemisia annua), and is usually used against malaria as part of a combination therapy. “Artemisinin kills malaria-causing parasites super fast—it will wipe out 90 percent of parasites within 24 hours,” says former postdoctoral researcher and co-first author Clare Harding, now a research fellow at the University of Glasgow. Once the fast-acting drug clears out the bulk of the parasites—such as Plasmodium falciparum, the culprit in the deadliest forms of malaria—from the bloodstream, the second drug finishes off the stragglers, curing the infection.
“Artemisinin works differently than most antibiotics,” Lourido said. “You can think of it as a sort of bomb that needs to be turned on in order to work.” The molecule required to light the drug’s fuse is called heme. Heme is a small molecule that facilitates several cellular functions, including electron transport and the delivery of oxygen to tissues as a component of hemoglobin. When heme molecules encounter artemisinin, they activate the drug allowing the creation of small, toxic radicals which react with proteins, lipids and metabolites inside the parasite, leading to its death.
Lourido, Harding, and co-first authors Boryana Petrova and Saima Sidik (“We were the ‘Heme Team,’” Harding said) wanted to understand what mechanisms the less susceptible parasites were using to avoid activating the “bomb”. Previously, Lourido and his lab—which focuses on apicomplexan parasites, a group which includes both Toxoplasma gondii and the malaria-causing Plasmodium falciparum—had developed a method to screen the entire genome of T. gondii to discover beneficial and harmful mutations. For a number of reasons, the screen does not work on Plasmodium parasites, but Lourido hypothesized that the related parasites’ genomes were similar enough that the method could prove helpful.
After running the screen, two genes stood out to the researchers as important factors in the parasites’ susceptibility to artemisinin treatment. One, called Tmem14c, seemed to be protecting the parasites: when the gene was disrupted in the screen, the parasites became more susceptible to treatment with artemisinin. The gene is analogous to one in red blood cells that serves as a transporter for heme and its building blocks, shuttling them in and out of the mitochondrion.
“What could be happening here is that, in the absence of Tmem14c, heme, artemisinin’s activator, collects within the mitochondria where it is being synthesized, thereby rendering the mitochondria better at activating that ticking time bomb,” Lourido said. “Having that high concentration of heme in the mitochondria is like having a flame when there is a gas leak.”
The screen also identified one mutation that led to parasites being less sensitive to artemisinin. The mutation affected a gene called DegP2, the product of which interacts with several mitochondrial proteins and appears to play a role in heme metabolism. When less DegP2 was present, the cells contained a lower amount of heme, which in turn made it less likely that the parasites would be killed by artemisinin.
Both the findings support other research suggesting that heme metabolism is crucial for artemisinin susceptibility. “It is important to consider the role of heme when combining artemisinin with other therapies,” Lourido said. “You would want to avoid combination therapy that might inadvertently suppress the level of heme within the parasite and thereby reduce susceptibility to antiparasitic agents.”
The project also showed the potential of using the Toxoplasma screening method as a model to study other related parasites. The screen confirmed findings in Toxoplasma that had previously been shown in Plasmodium, suggesting that it could be a valuable tool in studying malaria and other diseases caused by apicomplexan parasites.
“Through the amazing screens and molecular biology that you can do in Toxoplasma, we can really learn a lot about the biology of this diverse group of parasites,” Lourido said. “Defeating malaria is going to take a lot of different and creative approaches, and the fundamental research that we can do in Toxoplasma can in fact inform many of the critical clinical questions we need to answer to control this disease.”
***
Written by Eva Frederick
***
Harding, C., Sidik, S, and Petrova, B., et al. “Genetic screens reveal a central role for heme metabolism in artemisinin susceptibility.” Nature Communications. DOI: https://doi.org/10.1038/s41467-020-18624-0