Author: Raleigh McElvery
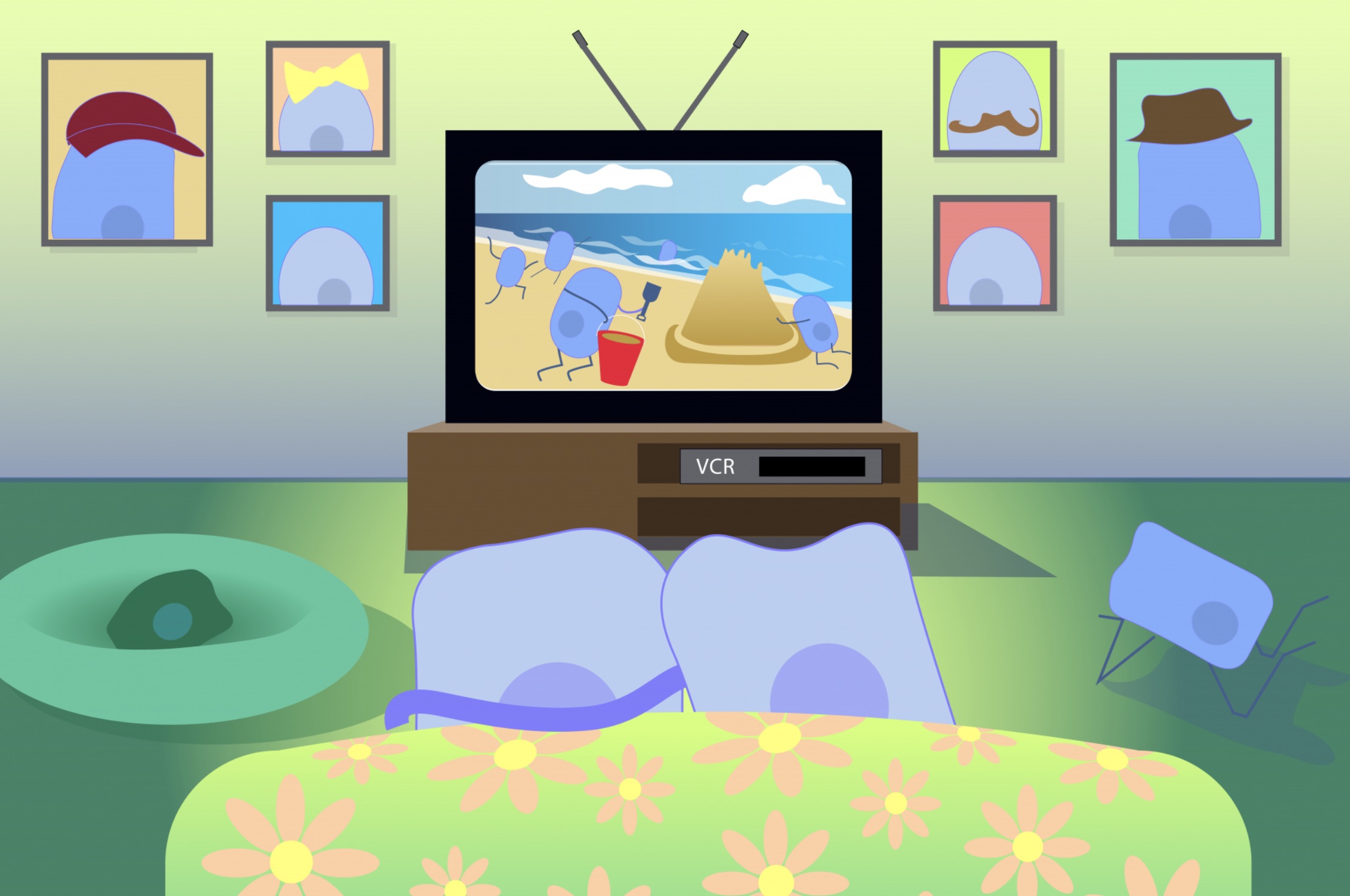
Whitehead Institute
February 9, 2021
Important moments of cancer — mutation, tumor formation, metastasis — are fleeting, easy-to-miss events. Even with modern medical technologies and methods, they often happen unobserved, and are only realized later when these cells spawn life-threatening conditions.
In recent years, however, new methods of tracking individual cells through time have allowed researchers to get closer to the origin of these events, and Whitehead Institute scientists are turning the power of these technologies to study cells involved in several different types of cancer. “With [these technologies], you can track down rare events in the past, identify all the offspring of an event, and see how they’ve changed their behavior,” said Whitehead Institute Member Jonathan Weissman. “You can ask, when a cell picks up an oncogene, how does it mutate, and further evolve, and proliferate and metastasize?”
Read on to learn how three Whitehead Institute Members are using specially engineered mice, CRISPR-based technologies, and other methods to track cells at different stages of cancer development, pushing the boundaries of what we understand about how the disease starts and proliferates. From the initial beginnings of a tumor, sometimes in the darkness of a still-forming embryo, to a tumor’s growth and eventual metastasis to other sites in the body, Whitehead Institute scientists Rudolf Jaenisch, Jonathan Weissman, and Robert Weinberg study the pivotal points in a cancer’s growth and spread.
The birth of a tumor
For around 800 children each year in the United States, the seeds are sown during fetal development for a rare and unpredictable childhood cancer called neuroblastoma. To understand how the disease develops, scientists need to study what happens to these cancerous “seeds” as the embryo matures. But they can hardly study a living human embryo, and fetal development is such a complex process that it is near impossible to simulate it in the lab.
To make matters more complex, the cancer grows and then may shrink unpredictably after the children are born and as they age. Sometimes the tumors disappear on their own; other times they grow uncontrollably.
In 2020, Institute Founding Member Rudolf Jaenisch’s lab introduced a new way of tracking the cells involved in the disease using his tried and true method for modeling such complex conditions: chimeric mice. A chimera is a conglomeration of two species — in this case, mostly mouse, with a few strategically placed human stem cells.
To create chimeras to study neuroblastoma, Jaenisch, who is also a Professor of Biology at Massachusetts Institute of Technology (MIT), along with collaborators at the Koch Institute for integrative Cancer Research at MIT and the Dana-Farber Cancer Institute, engineered human stem cells with glowing proteins to make them easy to see under the microscope. The cells contained a special genetic-switch that allowed the researchers to induce tumors by adding a certain chemical. These human stem cells were then induced to form a more specialized cell type – a neural crest stem cell.
Neural crest cells are a group of developing stem cells that go on to form the peripheral nervous system as well as other parts of the body such as the facial bones. It is neural crest cells that mutate into neuroblastoma tumors in humans, so the researchers hoped that by using these cells, they could create “human” tumors in mice. The researchers injected these human neural crest cells into mice so that they could readily incorporate with the host’s cells.
After the mice were born, the researchers were then able to take samples from the mice over the course of their development to see whether these implanted cells would form neural crest-derived tumors and, if so, what happened as they grew — something they would never have been able to do with neuroblastoma tumors in human infants and children.
The tumors in the chimeric mice pups developed in similar forms to human neuroblastomas — specifically, they formed characteristic rosette shapes — very similar to those seen in patient’s samples. With the help of Stefani Spranger, an assistant professor of biology at MIT and a Member of the Koch Institute for Integrative Cancer Research at MIT, they were able to track the cells’ interactions with the mice’s immune systems, and learn how the cancer “tricks” the immune cells into letting it stick around.
Now that they are able to model the formation of neuroblastoma tumors, the researchers hope to find a way to eliminate the tumors in the mice. “This is a model that will allow us to approach how to get rid of the tumors,” said Malkiel Cohen, a former postdoc in Jaenisch’s lab and first author of the paper, published in the journal Cell Stem Cell describing the work.
Cancer genealogy
A recent project from the lab of Whitehead Institute Member Jonathan Weissman focuses on another essential moment of cancer progression: metastasis. Metastasis happens when cancer spreads from a primary tumor to distant places in the body. Weissman, an expert in genome editing, created a CRISPR-based method to track the lineage of individual cancer cells in real time as they proliferate and metastasize.
Weissman and his collaborators, including graduate student Matthew Jones and then postdoctoral researcher Jeffrey Quinn, adapted the technology from a similar tool designed by Michelle Chan, a former postdoc in Weissman’s former lab at the University of California, San Francisco (UCSF) who is now an assistant professor at Princeton University. Chan designed a CRISPR mechanism to track the lineages of embryonic cells as they developed into specialized tissues.
“What Michelle Chan was able to do was uncover how tissues that look very similar to one another, actually come from disparate sources,” said Matthew Jones, a graduate student in Weissman’s lab and a co-first author on the paper describing the new method. “That has rapid implications about how tissues organize themselves, and we wanted to apply it to cancer.”
To create this system, the researchers engineered cancer cells with added genes: one for Cas9, the gene that codes for CRISPR’s “molecular scissors,” others for fluorescing proteins for microscopy, and a few sequences that would serve as targets for the CRISPR technology.
They then implanted thousands of these cells into the lungs of mice to simulate a tumor, using a model designed by Trever Bivona, a cancer biologist at UCSF. As the cells in the model tumors began to divide, the Cas9 protein began making small snips in the target sites in the cells’ DNA. When the cells fixed these snips, they patched in or deleted a few random nucleotides, leading to a unique “barcode” in each cell. Because these barcodes were added to each cell’s DNA, they were heritable and able to be passed on through generations of cells.
With the help of Nir Yosef, a computer scientist at the University of California, Berkeley, the researchers organized the data into “family trees” of cancer cells spanning multiple generations. By taking samples from different areas of the body, the researchers were able to resolve exactly when a cell jumped from where it started, in the lungs, to a distant tissue.
When they compared the trees, the researchers noticed that some cells were highly metastatic, jumping around multiple times over the course of the experiment, while others stayed put throughout. “We were excited to uncover some of these really rare, but consequential events that happened in the past that you would never be able to observe, and rarely be able to infer from a static snapshot,” Jones said.
By comparing highly metastatic and non-metastatic cells, they were able to identify metastasis-associated genes and answer questions about how the tumors were evolving and adapting. “It’s an entirely new way to look at the behavior and evolution of a tumor,” Weissman said. “We think it can be applied to many different problems in cancer biology.”
The next steps
A natural tumor begins with a single cell, mutated in a way that leads it to “go rogue,” so to speak. To mimic this in a model system, Dian Yang, another post-doc in the Weissman Lab, is collaborating with researchers in the lab of MIT Professor of Biology and Koch Institute Director Tyler Jacks’ to create a mouse model with the CRISPR lineage recording tool embedded in its DNA.
The model is based on a mouse model created by Jacks’ lab to study lung cancer. The lab has created genetically engineered mice that when left alone, live completely normal lives. But upon adding a trigger (an enzyme called “Cre”) — in Jacks’ and Yang’s case, they deliver the trigger to the lung using a virus — oncogenic signals are activated and lead to spontaneous tumors in the mice’s lungs.
Being able to “switch on” the mouse model in this way has a number of advantages. “Each tumor will start from one single transformed cell, which we can then watch in its native environment as it evolves,” Yang said. “Then, we can look back later at how the cells metastasize.”
Adding the CRISPR system to Jacks’ existing cancer model will also allow researchers to study cancerous cells on a broader time scale. “Usually, when we harvest samples from the mice, it is like a snapshot, just one sample at one stage,” Yang said. “You can see what it looks like, you can analyze gene expression at the time of sampling, you can even take a time series, but you don’t know what happened in the past.”
In a sense, the lineage recording technology embedded in the genome of the mice now makes it possible to look back in time. “When you have a million cells in a tumor, you can use the lineage network of these cells to find out how they’re related, and connect the current state with the past evolutionary lineage history,” he said. “I think this will provide a new dimension of biological information for us to understand biology that is not just limited to cancer biology.”The making of metastasis
Weissman’s lab’s method for tracking the lineage of cancer cells can illuminate the nature of the cells that leave the primary tumor and scatter throughout the body. But what actually happens to these cells to cause them to metastasize?
That’s where Whitehead Institute Founding Member Robert Weinberg’s research comes in. Weinberg has been studying cancer for decades. His early work helped to answer the question of how cells that form a primary tumor become cancerous. Weinberg identified the first human oncogene, a gene that causes otherwise-normal cells to form tumors. This finding, combined with others, demonstrated that cancer is a disease driven by damaged genes, at least in its origins. Weinberg has since turned his attention to the question of how cancer cells acquire the ability to spread.
Around 90 percent of cancer deaths are caused not by the primary tumor, but by its metastasis. Based on previous work, there is no single genetic switch that can be flipped to equip a cancer cell for metastasis. Instead, cells must go through a series of changes over time. Most cancer cells fail to acquire all of the necessary traits, and so may, for example, spread to new tissues but rarely form tumors there. Weinberg’s lab tracks cancer cells to help fill in the “road map” that cancer cells follow on the way to metastasis, in the hopes that their insights can be used to prevent or treat metastatic cancers.
One important change that cancer cells undergo is called the epithelial to mesenchymal transition (EMT), a cellular process that causes the cancer cells to express different genes and go from being immobile to mobile and invasive. Cancer cells undergoing this transition to be able to spread are called “quasi-mesenchymal.” The ability to spread does not fully explain metastasis, however.
“There’s two aspects of the metastasis problem,” Weinberg said. “The first aspect is how cancer cells physically leave the primary tumor and get seeded into a distant tissue. In other words, the physical translocation of the cells. The second step represents the subsequent ability of the already seeded cells to figure out how to make a living in a distant tissue.”
In other words, how do those transplanted cells adapt to a new tissue environment, which might offer them only inhospitable conditions? “That represents the major unsolved problem of metastasis,” he said.
Weinberg hopes to study this more in the future; for now though, his lab has found that studying quasi-mesenchymal cells can serve another purpose. Anushka Dongre, a postdoc in Weinberg’s lab, found that these cells are resistant to a common type of cancer treatment, known as immune checkpoint therapy, and can even protect the other cancer cells around them from that therapy. If as little as ten percent of a tumor consists of quasi-mesenchymal cells, then the whole tumor may become resistant.
By using a tumor’s epithelial/mesenchymal profile, Dongre demonstrated that she could predict how likely a breast cancer tumor was to respond to a particular immune checkpoint therapy. This finding could help physicians match patients to the best treatment plan, by indicating ahead of time whether the treatment will work. She also identified a way to eliminate the quasi-mesenchymal cells’ protective effect by suppressing a certain enzyme that they employ to defend themselves.
Weinberg’s lab continues to study pivotal changes in the lives of cancer cells, such as the EMT, so that they can better understand metastasis and, they hope, help find effective treatments for patients with metastatic cancers.Tracking cells into the future
Scientists have been tracking cells for more than a century, and Whitehead Institute scientists will be tracking cancer cells for decades to come. In the coming years, Weinberg plans to continue to investigate the mysteries of metastasis. For Weissman’s part, he hopes to continue refining his CRISPR technique, with the end goal of eventually being able to predict the behavior of cancer cells. “We want to be able to measure where they are, where they’re going at any time, and then predict where they’re going to be in the future,” he said.
With new technologies and ever-expanding fields, there is limitless potential in these various methods. “That’s what is so exciting about the cell tracking field right now,” said Matt Jones. “It’s really pushing the boundaries on what we can capture from our measurements.”
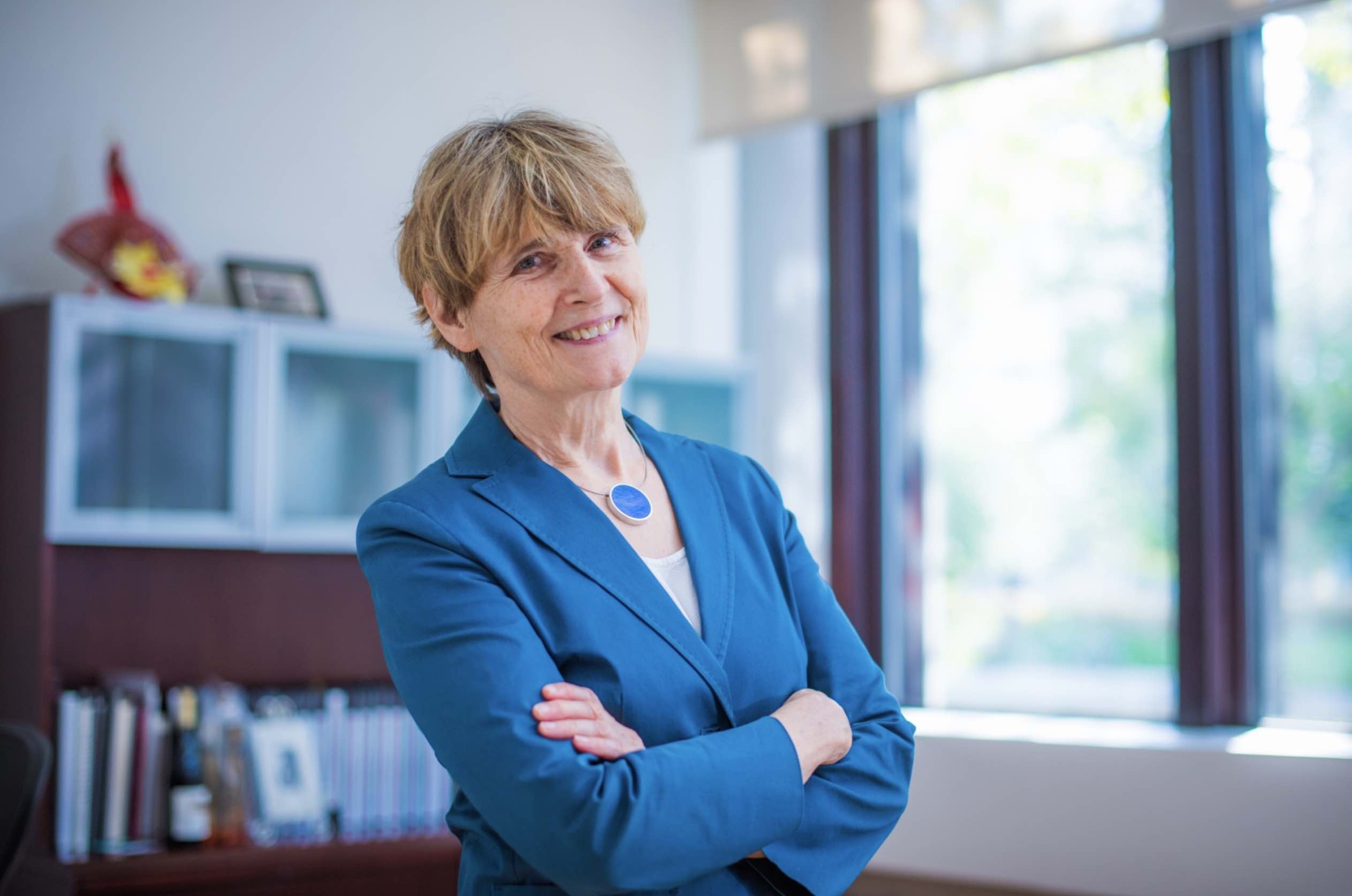
Whitehead Institute
January 27, 2021
Whitehead Institute director and Member Ruth Lehmann is the 2021 recipient of the Genetics Society of America’s (GSA) Thomas Hunt Morgan Medal for lifetime contributions to the field of genetics. The GSA is an international community of more than 5,000 scientists; and the Morgan Medal is one of the most prestigious awards for career achievement in the field of genetics.
The Morgan Medal was bestowed in recognition for Lehmann’s groundbreaking work revealing the unique biology of the specialized cells that give rise to egg and sperm. Known as germ cells, they are the only cells in the body with the power to build a new organism and transmit this potential to future generations. Lehmann’s research using the fruit fly Drosophila melanogaster has uncovered the molecular mechanisms by which germ cells are distinguished from the other cells of the body and how they migrate into position during development of the embryo. These and other highly influential discoveries from the Lehmann lab have spawned insights into many aspects of animal development, cell migration, cell signaling, RNA regulation, genome integrity, and mitochondrial inheritance.
This is the second consecutive year that a Whitehead Institute Member has received the Thomas Hunt Morgan Medal. It was awarded in 2020 to Whitehead Founding Member and former director Gerald R. Fink.
The Davis and Berger labs combined cryo-electron microscopy and machine learning to visualize molecules in 3D.
February 4, 2021
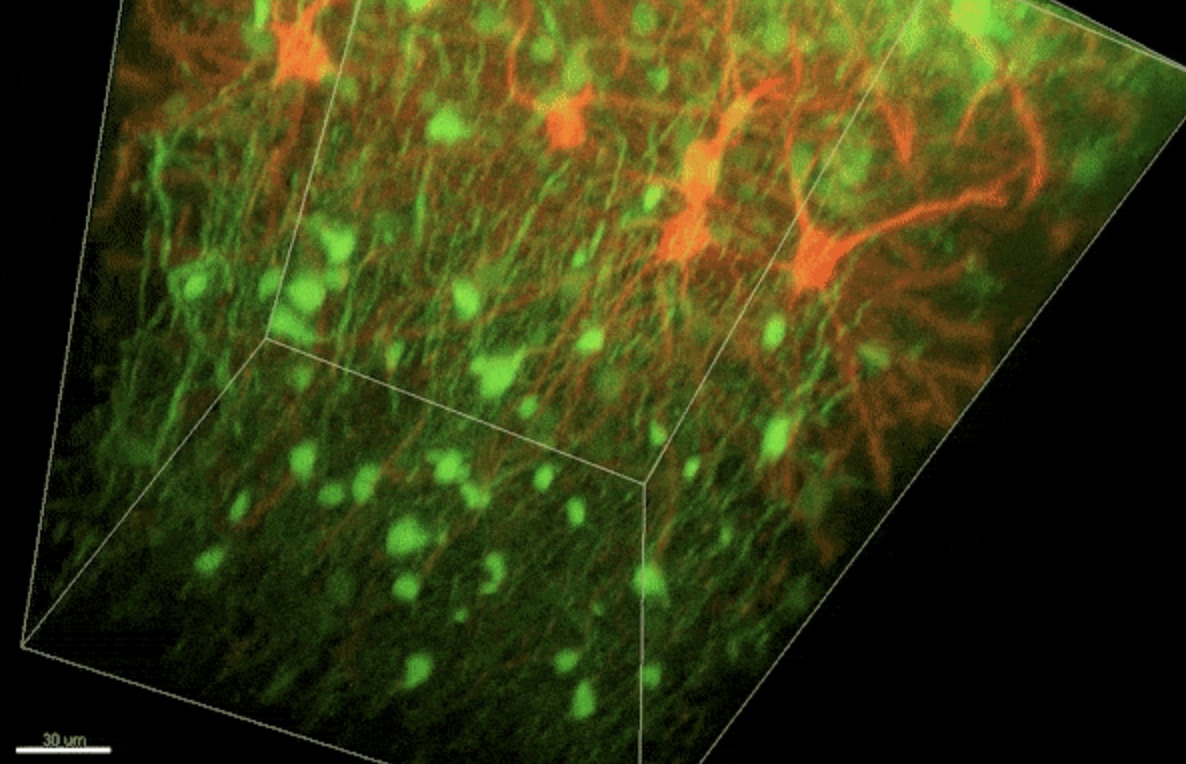
Neurons dynamically control their myelin patterns.
Picower Institute
January 1, 2021
Harvard and MIT researchers have discovered a new way that the brain responds to stimuli, with different types of neurons using myelin in different ways. By dynamically controlling myelin, the insulating coating around their long axon projections that helps with signal conduction, neurons have more ways to adapt to changes. Published in the journal Science, the study in mice advances scientists’ understanding of how the brain works and opens avenues for exploring new disease mechanisms.
“The brain requires a diversity of mechanisms at its disposal in order to adapt to stimuli. We know that neurons have different properties, and we demonstrated several years ago that neurons have different patterns of myelination. Now, we have found an additional layer of complexity: neurons actively use their myelin in dynamic and different ways,” said co-senior author Paola Arlotta, the Golub Family Professor of Stem Cell and Regenerative Biology at Harvard University.
In biology textbooks, the prototypical image of a neuron depicts the axon with a series of equally sized, evenly spaced pieces of myelin. However, the Arlotta lab showed in 2014 that the picture is more complicated: different types of neurons show different patterns of myelination, with varying lengths of myelin or no myelination on some segments. In the current study, the researchers delved deeper into the phenomenon of how myelination patterns might change over time.
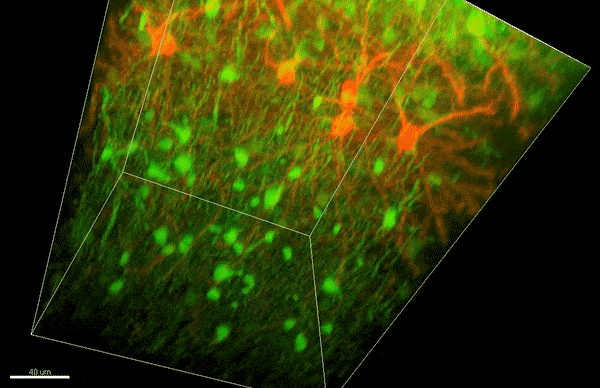
To investigate myelin plasticity, the researchers used mouse models where specific neuron types were fluorescently labeled. They changed the animals’ sensory input by closing one eye, then tracked how the brain responded using a custom-built in vivo imaging system in the lab of collaborator and co-senior author Elly Nedivi.
“Our multicolor method enables the simultaneous visualization of both the myelin and the axons it was wrapping. This allowed us to closely track how myelin was changing over time as mice reconfigured the visual cortex as sight became deprived in one eye,” said Nedivi, who is the William R. (1964) & Linda R. Young Professor of Neuroscience at MIT, and a member of The Picower Institute for Learning and Memory and the Departments of Biology and Brain and Cognitive Sciences.
The researchers found that even though they tracked neurons that were next to each other and part of the same network, different cell types had different responses — specifically, inhibitory neurons remodeled their myelin more than excitatory neurons.
“In the inhibitory neurons, we saw a two-times increase in the number of myelin changes. Those changes can be any way you can imagine: they can be myelin segments shortening or elongating, the addition of new myelin, and also the elimination of an entire piece of myelin,” said Sung Min Yang, lead author and postdoctoral fellow in the Arlotta lab.
The unique capacity to change their myelin opens up possibilities for the neurons, Yang said: “It turns out that neurons do not move myelin around in a consistent way, as in a game of checkers where every game piece has the same move. Instead, the brain is playing chess, where different neurons — or pieces — can move in different ways. This gives the brain more choice in how to use myelin, which is a limited resource.”
The researchers also found that neurons did not necessarily have to produce new myelin, but could reuse and reshape what they already had in order to respond.
“We have discovered a fundamental property of the brain that opens the window to conceptualizing how the organ maximizes its power and optimizes its function. The dynamic distribution of myelin is yet another level of mechanism that the brain uses to diversify its response to a given stimulus — the endless combinations can enable a more complex, even surprising outcome,” Arlotta said.
Based on the findings, the researchers can now investigate how myelin plasticity plays a role in other contexts, including disease.
“We hope to be able to investigate myelin pathology in human brain organoid models, which can be generated from patients or engineered to contain specific mutations associated with myelin abnormalities, in order to better understand the disease mechanisms,” Arlotta said.
This research was supported by the Stanley Center for Psychiatric Research at the Broad Institute of MIT and Harvard, the National Institute of Mental Health, the National Institutes of Health, and the JPB Foundation.
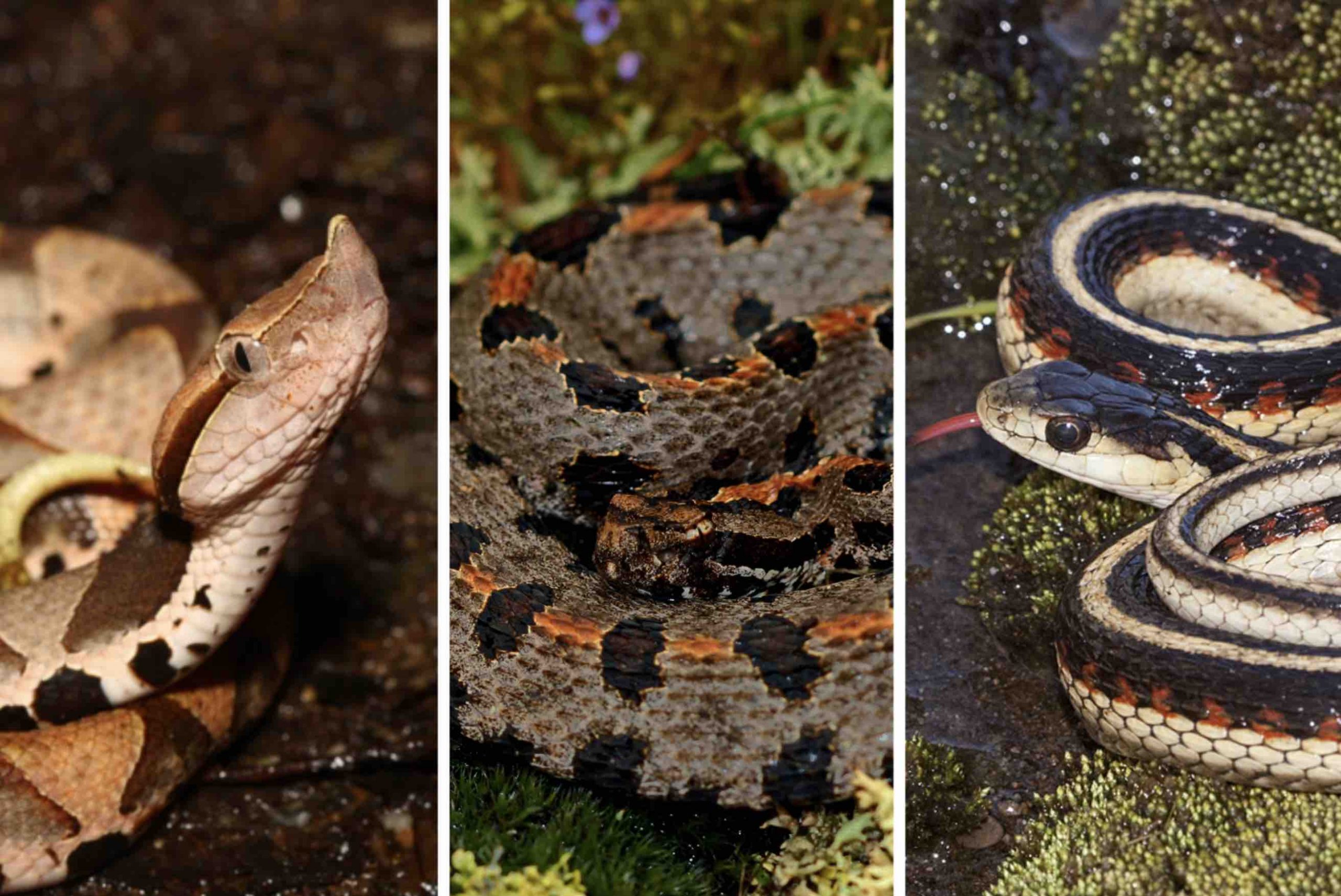
Eva Frederick | Whitehead Institute
January 21, 2021
Sex-specific chromosomes are a dangerous place to be, if you’re a gene. Because these chromosomes — Y chromosomes, in humans — do not have a matching chromosome with which to exchange genetic information, they are prone to losing non-essential genes left and right in a process called genetic decay.
Now, a new study from research scientist Daniel Winston Bellott in the lab of Whitehead Institute Member David Page broadens our understanding of what makes a gene able to survive on a sex-specific chromosome by looking at one especially slithery branch of the evolutionary tree: snakes.
Comparing surviving genes on snake sex-specific chromosomes to those that are lost to the ravages of time can teach scientists about the evolutionary pressures that shaped sex chromosomes as we know them today. “You might think, ‘These are sex chromosomes, so the surviving genes should have something to do with sex, right?’” Bellott said. “But they don’t.”
Instead, many of these genes are essential to the survival of the animal, and take part in key developmental processes. “It turns out that these survivor genes on sex-specific chromosomes may play a very big role in governing how all of the genes across all the chromosomes are read, interpreted and expressed,” said Page, who is also a professor of biology at Massachusetts Institute of Technology (MIT) and an Investigator of the Howard Hughes Medical Institute. “Winston’s study is absolutely foundational to our understanding of what the sex chromosomes are, how the two sexes come to be, and how health and disease traits play out similarly or differently in males and females.”
What is a sex chromosome, anyway?
Over the course of evolution, all sex chromosomes start out as regular, matching chromosomes called autosomes. Then, somewhere along the line, a mutation happens, and one of the chromosomes gains a “switch,” that, when present, causes an embryo to to develop as a specific sex. “It’s actually really easy to make a sex chromosome,” said Bellott. “In most cases, you only need to change one or two genes and you’ve started the sex chromosome system.”
This process has happened numerous times during the course of evolution. It makes sense; sexual reproduction is an efficient way to ensure genetic diversity. But the whole thing is a bit mysterious; are, for example, certain chromosomes predisposed to become sex chromosomes?
That’s where Bellott thought snakes could be especially helpful. “Snakes have a relatively old system of sex chromosomes, where you have a lot of time for the chromosomes to diverge,” Bellott said. “Time has swept away all the genes that aren’t important, and you can see what kind of genes are left.”
Their sex chromosome system also evolved from different autosomes, some 100 million years after humans’, and thus would provide a useful vantage point from which to consider our own genomes.
To learn more about the evolution of these chromosomes, Bellott and Page first gathered a list of “ancestral genes,” which were likely on the chromosome from which the snake sex chromosomes evolved. New sequencing data for several species of animals distantly related to snakes meant that they had a more complete list of these genes — 1,648 to be exact.
Bellott began painstakingly sifting through the genes that remained on the sex-specific chromosomes of three species of snake: the pygmy rattlesnake, mountain garter snake, and the five-pacer viper. He eventually identified 103 ancestral genes that had survived as long as 90 million years of evolution on the snakes’ sex chromosomes. With this list in hand, Bellott could then ask what these surviving genes had in common that set them apart from the hundreds of genes that were swept off the snakes’ sex chromosomes by genetic decay.
What makes a survivor?
To Bellott’s surprise, the genes that remained on the snakes’ sex-specific chromosome had nothing to do with sex determination; neither were they expressed more often in sex-specific tissues, or more often in one sex than the other.
Instead, Bellott and Page’s research identified three key properties that led to a gene’s survival on snake sex-specific chromosomes. First, the gene must be dosage sensitive. In other words, the snake’s body depends on its cells to produce an exact amount of that gene’s protein product. Any more, or any less, and the snake experiences illness or death. Second, a surviving gene is likely broadly expressed in different tissues across the body, not localized to one specific organ or area. And third, surviving genes are subject to strong purifying, or negative, selection. Simply put, this means that if something goes wrong with one of these genes, the snake has a slim chance of survival or producing offspring.
When Bellott dove deeper into the genes’ function, he discovered that for many of them the equivalent gene in humans played a role in key developmental processes such as the formation of the face. When these genes were mutated in humans, their faces — and other essential parts of the body — would not develop properly. “What Winston is seeing here is that the genes that were preserved on the sex specific chromosomes in snakes are disproportionately involved in birth defects in people,” Page said. “We think that nature is selecting for the survival of [sex chromosome] genes whose dosage in certain parts of embryonic development is especially critical.”
In time, Bellott said, this may allow scientists to predict genes whose role in developmental disorders is yet to be discovered. “In some sense, you get to the place where you’re starting to work the experiment backwards in your mind, and say, ‘Let’s take the set of genes that are on sex specific chromosomes in snakes and birds, but that have not yet been implicated in birth defects in humans,’” Page said. “They might be prime candidates to be responsible for heretofore unexplained birth defects.”
From snakes to humans
Next, the researchers sought to broaden their scope. They compared ancestral genes across the three species of snakes and 38 species of birds and mammals with a larger pool of genes that made it to the present day. Many of the surviving genes on bird and mammal chromosomes had different functions than those on snake chromosomes, but again, most had little to do with sex determination.
“Adding the snakes in with the birds and the mammals gave Winston enough data points to be able to see further and to see more precisely, and now for the first time, he was able to confirm something that we had been suspecting for a long time but really didn’t have sufficient data to pin down,” Page said. “And that is that the chromosomes that became sex chromosomes were not sort of inclined to function in sex differences. Before they got picked out of the crowd, they weren’t specialized towards differentiating between the sexes in much of any way.”
Then, as genes were lost over time, evolutionary pressures ensured that the same sort of genes survived. This idea that sex chromosomes — besides their key developmental switch — have little do do with sex determination challenges the common notion of what a sex chromosome actually is.
“I hope people will pick up on this idea that the chromosomes that became sex chromosomes weren’t in any way preordained,” Page said. “They were just ordinary chromosomes out for a walk in the park, and something happened.”
In the future, Bellott and Page plan to further broaden their scope to include other animals, toward the ultimate goal of understanding our own sex chromosomes. “We take these results, and we turn them into a lens through which we look at sex differences in health and disease in our own species,” Page said. “This research really refines our ideas about what it means to be a gene on the human X or Y chromosome, and how we should think about those genes that survive.”
Bellott, D.W. and Page, D.C. “Dosage-sensitive functions in embryonic development drove the survival of genes on sex-specific chromosomes in snakes, birds, and mammals.” Genome Research, Jan. 21, 2021. DOI: 10.1101/gr.268516.120.
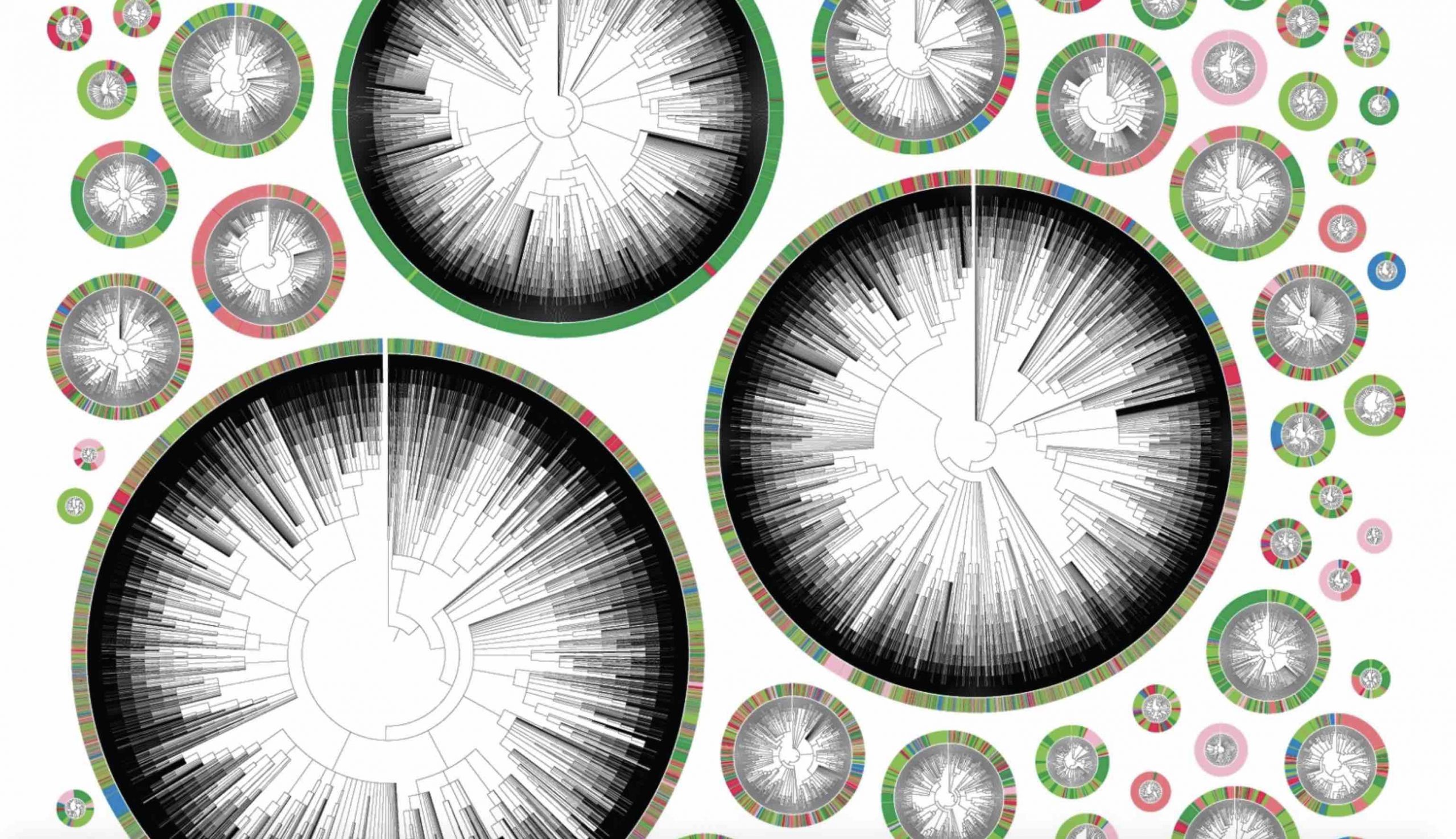
Eva Frederick | Whitehead Institute
January 21, 2021
When cancer is confined to one spot in the body, doctors can often treat it with surgery or other therapies. Much of the mortality associated with cancer, however, is due to its tendency to metastasize, sending out seeds of itself that may take root throughout the body. The exact moment of metastasis is fleeting, lost in the millions of divisions that take place in a tumor. “These events are typically impossible to monitor in real time,” said Whitehead Institute Member Jonathan Weissman.
Now, researchers led by Weissman, who is also a professor of biology at Massachusetts Institute of Technology and an investigator with the Howard Hughes Medical Institute, have turned a CRISPR tool into a way to do just that. In a paper published January 21 in Science, Weissman’s lab, in collaboration with Nir Yosef, a computer scientist at the University of California, Berkeley, and Trever Bivona, a cancer biologist at the University of California, San Francisco (UCSF), treats cancer cells the way evolutionary biologists might look at species, mapping out an intricately detailed family tree. By examining the branches, they can track the cell’s lineage to find when a single tumor cell went rogue, spreading its progeny to the rest of the body.
“With this method, you can ask questions like, ‘How frequently is this tumor metastasizing? Where did the metastases come from? Where do they go?’” Weissman said. “By being able to follow the history of the tumor in vivo, you reveal differences in the biology of the tumor that were otherwise invisible.”
Scratch paper cells
Scientists have tracked the lineages of cancer cells in the past by comparing shared mutations and other variations in their DNA blueprints. These methods, however, depend to a certain extent on there being enough naturally occurring mutations or other markers to accurately show relationships between cells. That’s where Weissman and co-first authors Jeffrey Quinn, then a postdoctoral researcher in Weissman’s lab, and Matthew Jones, a graduate student in Weissman’s lab, saw an opportunity to use CRISPR technology — specifically, a method developed by Weissman Lab member Michelle Chan to track embryo development — to facilitate tracking. Instead of simply hoping that a cancer lineage contained enough lineage-specific markers to track, the researchers decided to use Chan’s method to add in markers themselves. “Basically, the idea is to engineer a cell that has a genomic scratchpad of DNA, that then can be ‘written’ on using CRISPR,” Weissman said. This ‘writing’ in the genome is done in such a way that it becomes heritable, meaning a cell’s grand-offspring would have the ‘writing’ of its parent cells and grandparent cells recorded in its genome.
Notes
Jeffrey J. Quinn, Matthew G. Jones, Ross A. Okimoto, Shigeki Nanjo, Michelle M. Chan, Nir Yosef, Trever G. Bivona, Jonathan S. Weissman. “Single-cell lineages reveal the rates, routes, and drivers of metastasis in cancer xenografts.” Science, Jan. 21, 2021.
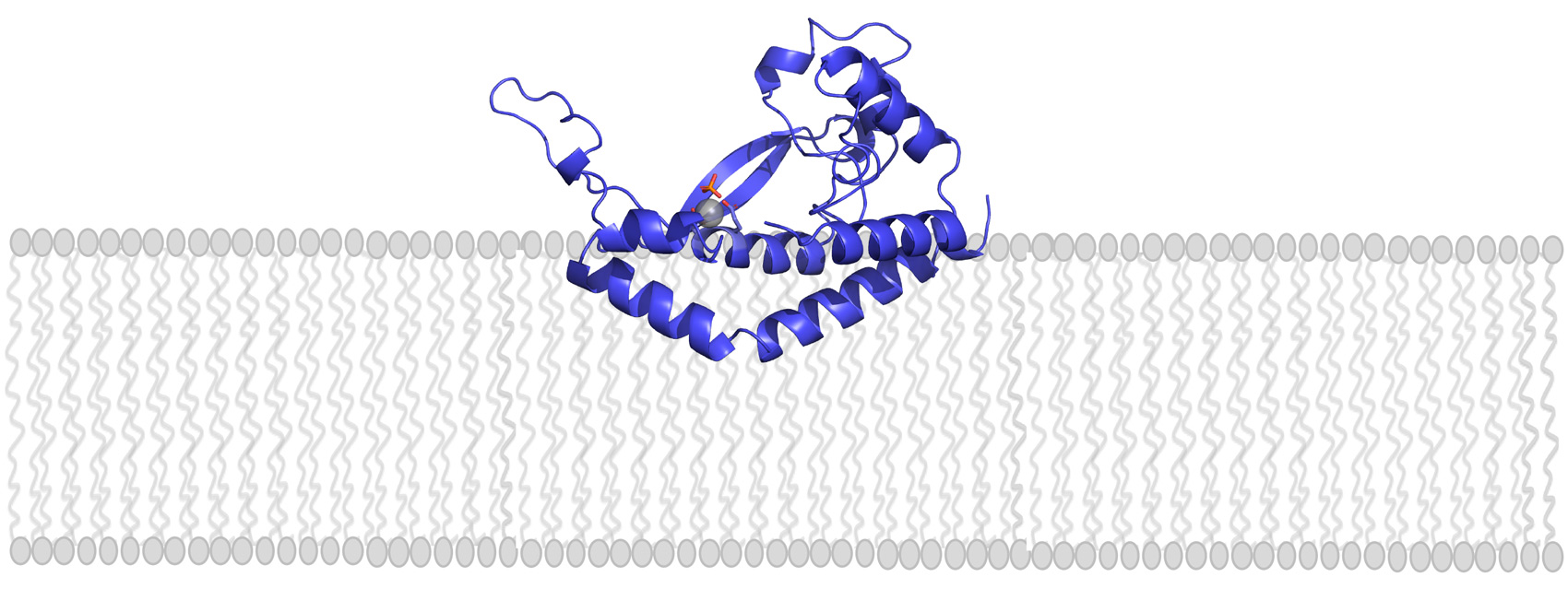
Raleigh McElvery
January 20, 2021
Many cells, including bacteria, are covered in a sugar-rich coating that protects their membrane and internal components. These sugars are often joined to other macromolecules, like proteins or lipids, to form glycoconjugates. The glycoconjugates that encrust bacteria help prevent them from “popping” under environmental stress, and facilitate host-pathogen interactions. Because the sugary layer perpetuates survival and virulence, researchers are looking for ways to create chinks in this microbial armor — or better yet, to prevent it from being made in the first place.
Glycoconjugates are built by many enzymes working in close succession at the cell membrane. One enzyme family, comprised of phosphoglycosyl transferases (PGTs), is responsible for catalyzing the first step in the assembly line. Of this large enzyme family, one subtype in particular stands out: “monotopic” PGTs, which are unique to bacteria and could serve as antibiotic targets. If researchers can develop drugs that inhibit monoPGTs, the sugar armor wouldn’t be built and noxious bacteria could be easier to defeat.
A new PNAS study co-authored by Professor of Biology and Chemistry, Barbara Imperiali, highlights the diversity and significance of these potential drug targets. Imperiali teamed up with graduate student Katherine O’Toole and Professor of Chemistry Karen Allen from Boston University to categorize over 38,000 different monoPGTs, compiling this information into the first database of its kind.
“We’ve taken an enzyme family that was once considered quirky and insignificant, and demonstrated that it’s actually very prevalent,” Imperiali says. “Hopefully the database will help us better understand these enzymes, their molecular pathways, and the human pathogens they support.”
Imperiali and her colleagues used sequence analysis of known monoPGTs to define a “signature” amino acid sequence. They leveraged this signature to identify the entire superfamily of monoPGTs amidst the 63,152 sequences downloaded from an online portal, which they then clustered into closely-related subtypes. The researchers also created a family tree, which included over 100 monoPGTs from diverse bacterial species. Imperiali hopes others will take advantage of this new information to pinpoint monoPGTs in pathogens of interest, and explore similarities and differences in related microbes and their enzymes.
The researchers’ analyses also revealed strange, new proteins that appeared to include two enzymes in one — a monoPGT fused to one of the other enzymes that typically play a separate role in the same sugar-modifying pathway. “It’s essentially one protein with two functions,” Imperiali explains. These fusion enzymes could reveal which enzymes “talk” to one another and work sequentially during the glycoconjugate-building process, she adds, revealing the complicated chain of events that creates the bacterial sugar shield.
The team even found cases where one monoPGT was fused to a member of a different PGT family — polytopic PGTs (polyPGTs). MonoPGTs and polyPGTs are involved in different pathways that each build glycoconjugates, so having a dual-function protein could allow cells to easily switch between mechanisms. Bacterial cells lack the organizational compartments that human and other eukaryotic cells have, so perhaps these fusion enzymes help exert control and order at different points in the cell cycle, Imperiali speculates. At the moment, though, the hybrid PGTs remain an evolutionary mystery.
While some researchers parse these ancient puzzles, others may use the database to inspire new drugs to combat antibiotic resistance. “At the end of the day,” Imperiali says, “we’ve shed light on a set of enzymes that could become pivotal therapeutic targets.”
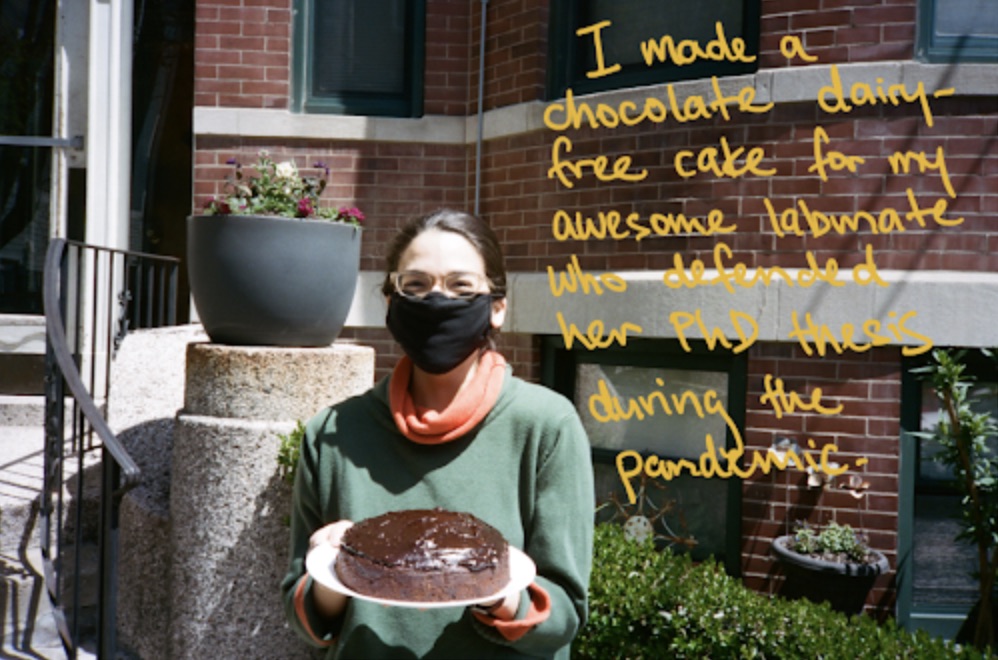
Irene Shih | MindHandHeart
January 13, 2021
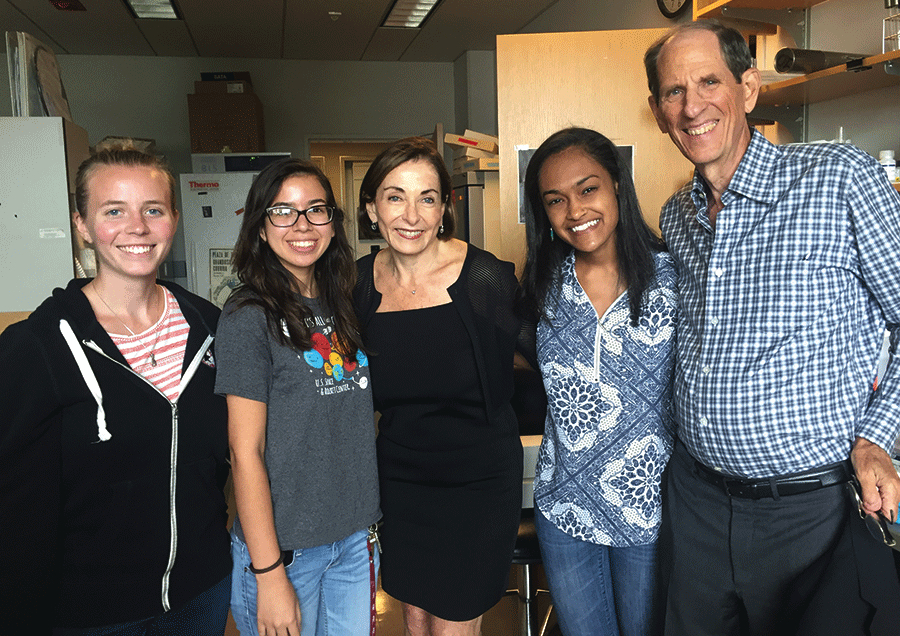
December 22, 2020
Dear colleagues,
I’m writing to share some really good news about our MIT’s Summer Research Program in Biology, or as most of us know it, MSRP-Bio. The simple take-home message: we now have endowed funds from Mike Gould and Sara Moss that will support about a dozen MSRP-Bio students each summer, for a very long time!
In 2015, Mike and Sara established the Bernard S. and Sophie G. Gould Fund to support students participating in MSRP-Bio. This gift was to provide opportunities to deserving students and to honor the memory of Mike’s parents.
Mike’s parents were both MIT alumni, and his father Bernie was a professor in the Biology Department from 1934 – 1987. Bernie and Sophie both committed their lives to supporting and counseling young students, and Mike and Sara chose to establish this fund to honor Mike’s parents and their deep and shared commitment to mentorship. Indeed, Mike and Sara share that commitment to support students and provide them with opportunities that could change their lives.
Mike and Sara have been remarkably dedicated to MSRP-Bio. Beginning with the first cohort of Gould Fellows in 2016, they visited each summer to meet and get to know these talented students. The first four meetings were in person, and then in the summer of 2020, the meeting was virtual due to the pandemic. Mike and Sara insisted on having the meeting, and even attended all of the student talks that summer. They have kept in touch with several of the Gould Fellow alumni and have gotten together with those who are in their hometown (NYC).
Mike and Sara have been so touched by the impact of their initial gift that they decided recently to provide additional support. To acknowledge this support and their commitment to our students and program, we are renaming MSRP-Bio the Bernard S. and Sophie G. Gould MIT Summer Research Program in Biology: BSG-MSRP-Bio, in honor of Mike’s parents.
We are deeply grateful to Mike and Sara for their commitment to and support for our community, their willingness to enable opportunities for students, irrespective of their specific research interests, and for the many talented individuals who will benefit from the experiences afforded by their generous gift.
This gift is a great way to end the year. Wishing everyone a wonderful holiday season and a Happy Healthy New Year!
Best wishes,
Alan Grossman, Department Head