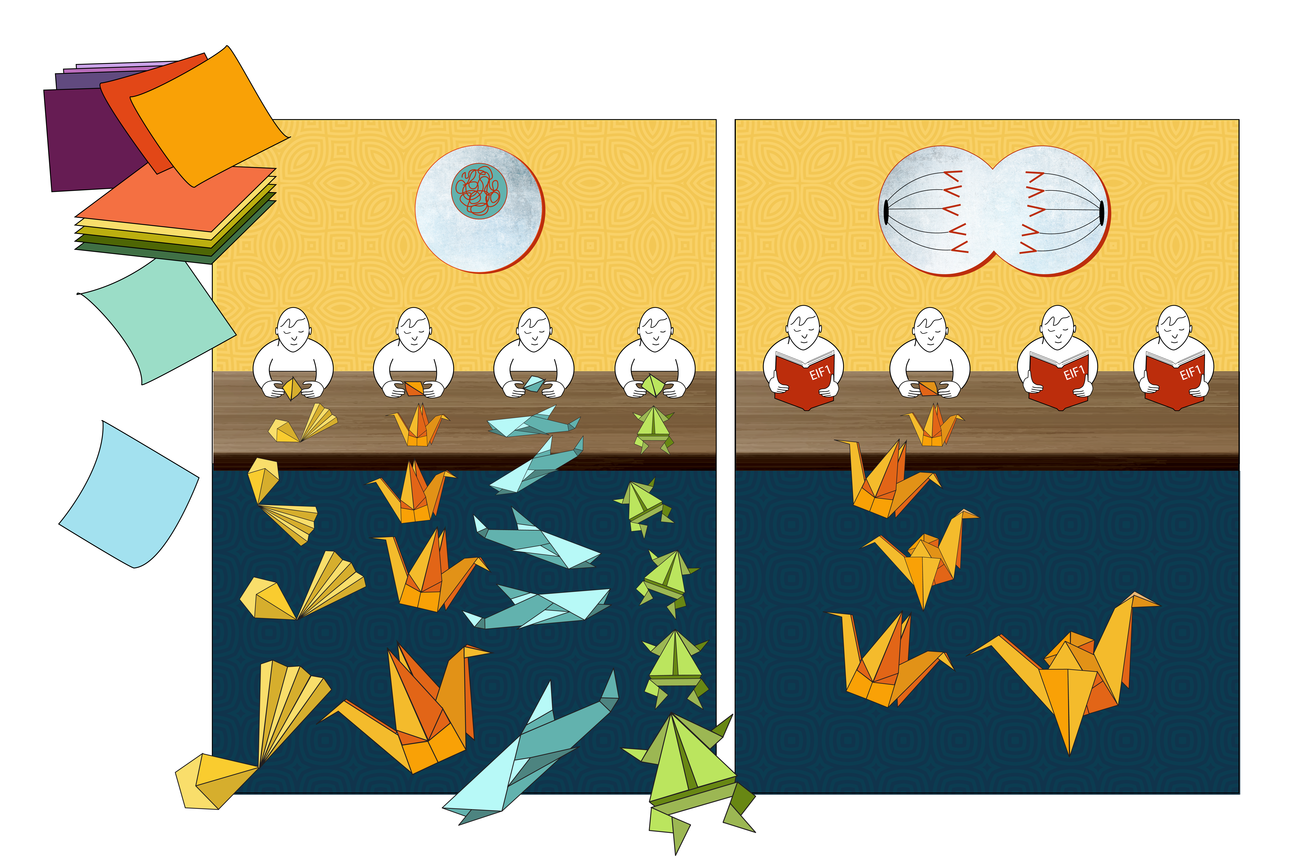
Cells make variants of thousands of proteins. These variants are not produced indiscriminately, but rather through precise regulatory mechanisms that can meet rapidly changing needs of the cell according to new research from the Cheeseman Lab.
Greta Friar | Whitehead Institute
October 18, 2024
Our cells contain thousands of proteins that have gone largely undetected and unstudied until recent years: these are variants of known proteins, which cells can make when their protein-building machinery interacts differently with the same stretch of genetic code. These protein variants have typically been overlooked as occasional accidents of gene expression, but researchers including Whitehead Institute Member Iain Cheeseman are discovering that they are actually abundant and can play important roles in cell functions. Researchers in Cheeseman’s lab are studying individual protein variants to learn more about them and their roles in health and disease, but they also wanted to understand broader patterns of protein variant production: how do cells control when to make one variant of a protein versus another, and what are the consequences of such switches?
Cheeseman, who is also a professor of biology at the Massachusetts Institute of Technology, and graduate student in his lab Jimmy Ly have now identified how cells switch to a different pattern of protein variant production during mitosis, or cell division. In research published in the journal Nature on October 23, they show that this broad regulatory switch helps cells survive paused cell divisions that can sometimes occur in healthy humans or be triggered by certain chemotherapy treatments. The work confirms that cells make variants of thousands of proteins, and also demonstrates that cells do not do so indiscriminately. Rather, cells use precise regulatory mechanisms to switch between different patterns of protein variant production, in order to rapidly tailor the proteins available to fit the changing needs of the cell.
A plethora of hidden proteins
Hw can our cells contain unknown proteins? In high school biology classes, students learn the rule that each gene codes for exactly one protein, such that if you know an organism’s genetic code, you should know every protein it can make. In fact, there are instead many genes that code for multiple proteins. For a protein to be made, first the genetic code for it is copied from DNA into a messenger RNA (mRNA). Then, a ribosome, the cellular machine that follows the instructions in genetic code to build a protein, locates the coding sequence within the mRNA by scanning for the start codon, a sequence of the three bases A, U, and G – bases are the chemical building blocks of RNA, abbreviated as A, U, C, and G. The ribosome recognizes the AUG start codon as the place to begin following instructions, and builds a protein based on the genetic sequence from there through to another trio of bases called a stop codon. However, one way that different versions of a protein can be produced is that a ribosome may begin reading the instructions from multiple different starting points.
Sometimes, a ribosome may miss the first AUG start codon and skip ahead to another AUG somewhere in the middle of the gene’s code, creating a truncated version of the protein. Sometimes, a ribosome may treat a similar trio of bases, such as CUG or GUG, as a start codon. This can cause it to begin earlier, creating a protein based on an extended genetic sequence. These possibilities mean that cells contain thousands more different proteins, or variants of proteins, than are represented by the dogma of one gene, one protein.
In order to understand protein variant production, the researchers—in collaboration with researchers from Whitehead Institute Member David Bartel’s lab–used a method that let them carefully track ribosomes to compare which start sites ribosomes tended to use. They looked at start site selection during mitosis versus during the rest of the cell cycle and found that a dramatic shift in use occurred for thousands of start sites. Specifically, the researchers found that during mitosis, ribosome scanning becomes more stringent. The ribosome will only begin making proteins at AUG sequences, and even then, only at AUGs that have preferable sequences of bases surrounding them—known as a strong Kozak context. This increased selectivity does not always lead to the familiar version of the protein being made during mitosis; sometimes the first AUG start codon has a weak Kozak context, so a truncated protein gets made from an AUG start codon with a stronger Kozak context that lies within the gene.
“Coming into this project, we knew very little about protein production during mitosis—for a long time, people didn’t think much protein production happened in mitosis at all,” Ly says. “It was satisfying to show not only that it is occurring, but that there’s a shift in which proteins are being made—and that this shift is important for cellular viability.”
How cells switch between protein variant programs
The researchers next identified how the switch to increased stringency is initiated during mitosis. They discovered that the key player is a protein called eIF1, which is one of many partners that can pair with ribosomes to help them select their start site. In particular, increased eIF1 pairing with ribosomes causes the ribosomes to be more stringent in their start codon selection, inhibiting the usage of non-AUG initiation sites or sites with weak Kozak contexts.
During mitosis, ribosome pairing with eIF1 increases sharply, leading to the shift in stringency. This change in pairing rate during mitosis puzzled the researchers: ribosomes and their partners, including eIF1, all typically reside together in the main body of the cell—where ribosomes make proteins—so they should be able to pair freely at any time. The researchers looked for other molecules in the same location that could be altering how ribosomes and eIF1 interact during different parts of the cell cycle, but they couldn’t find anything. Eventually, the researchers realized that the answer to the puzzle lay in a separate location: the nucleus.
They found that cells maintain a large pool of eIF1 inside of the nucleus, locked away from the ribosomes. Then, during cell division, the wall of the nucleus dissolves, mixing its contents with the rest of the cell. This is necessary for the dividing cell to divvy up its DNA, but it also releases the pool of eIF1 to pair with ribosomes, increasing stringency. At the end of mitosis, the nucleus reforms and eIF1 is re-incorporated into the nucleus of each of the two daughter cells, and the cells return to a less stringent program.
“The explanation for increased interaction between eIF1 and ribosomes during mitosis had really stumped us, and so when I saw eIF1 localizing to the nucleus, that was a really exciting ‘aha’ moment,” Ly says. “Discovering this mechanism of nuclear release during mitosis was unexpected, and it’s interesting to think about how else cells might be using it.”
Consequences of increased stringency for the cell
Once the researchers understood the how, they then wanted to understand the why? What they discovered is that when cells have no nuclear pool of eIF1, and so no change in stringency during mitosis, they are more likely to die during mitosis. In particular, these cells fare poorly during mitotic arrest, a state in which cells get stuck in mitosis for hours or even days–much longer than typical mitosis. Arrest occurs when cells detect a possible cell division error and so halt their division until the error is corrected or the cell dies.
One effect of increased stringency during mitosis is related to mitochondria, which are required for energy production in many cell types and are therefore required for maintaining viability. Cells stuck in mitotic arrest need energy to keep them going through this unexpected delay. The researchers found that increased stringency during mitosis led to an increase in the production of important mitochondrial proteins, boosting the cells’ energy supply to get them through arrest.
Increased stringency also gives cells the tools they need to escape arrest, even if they haven’t fixed the error that caused them to pause division. In a Nature paper in 2023, Cheeseman and then-postdoc in his lab Mary-Jane Tsang showed that when cells build up enough of the truncated version of a protein called CDC20, they can escape arrest. Ly’s work adds to this story by showing that the nuclear release of eIF1 increases stringency, leading to more production of truncated CDC20 during mitosis, which explains how cells build up enough of this protein variant during mitosis to trigger their escape. These findings may have important potential implications for some cancer chemotherapy strategies.
Some chemotherapies work by trapping cancer cells in mitotic arrest until they die. Cheeseman, Tsang, and Ly’s work collectively shows that when cancer cells lack sufficient truncated CDC20—as can occur in the absence of nuclear eIF1—the cells cannot escape arrest and so are killed off by these chemotherapies at higher rates. These results could be used to improve the efficacy of antimitotic chemotherapy drugs.
The switch in protein variant production that the researchers found affects thousands of proteins. These newly identified protein variants serve as a foundation for many future projects in the lab.
As the researchers continue to examine the consequences of this switch to stringency during mitosis, they are also searching for other cases in which cells regulate protein variant production outside of mitosis. For example, the researchers are interested in how this switch in stringency affects fertility; immature egg cells spend a long time in a form of arrested cell division without an intact nucleus, and Ly observed eIF1 in the nucleus of the immature female eggs.
“Cells have axes of control that they use to quickly make broad changes in gene expression,” Cheeseman says. “Several of these are central to controlling cell division—for example, the role of phosphorylation as a regulatory switch in mitosis has been well studied. Our work identifies another axis of control, and we’re excited to discover more about when and how cells make use of it.”