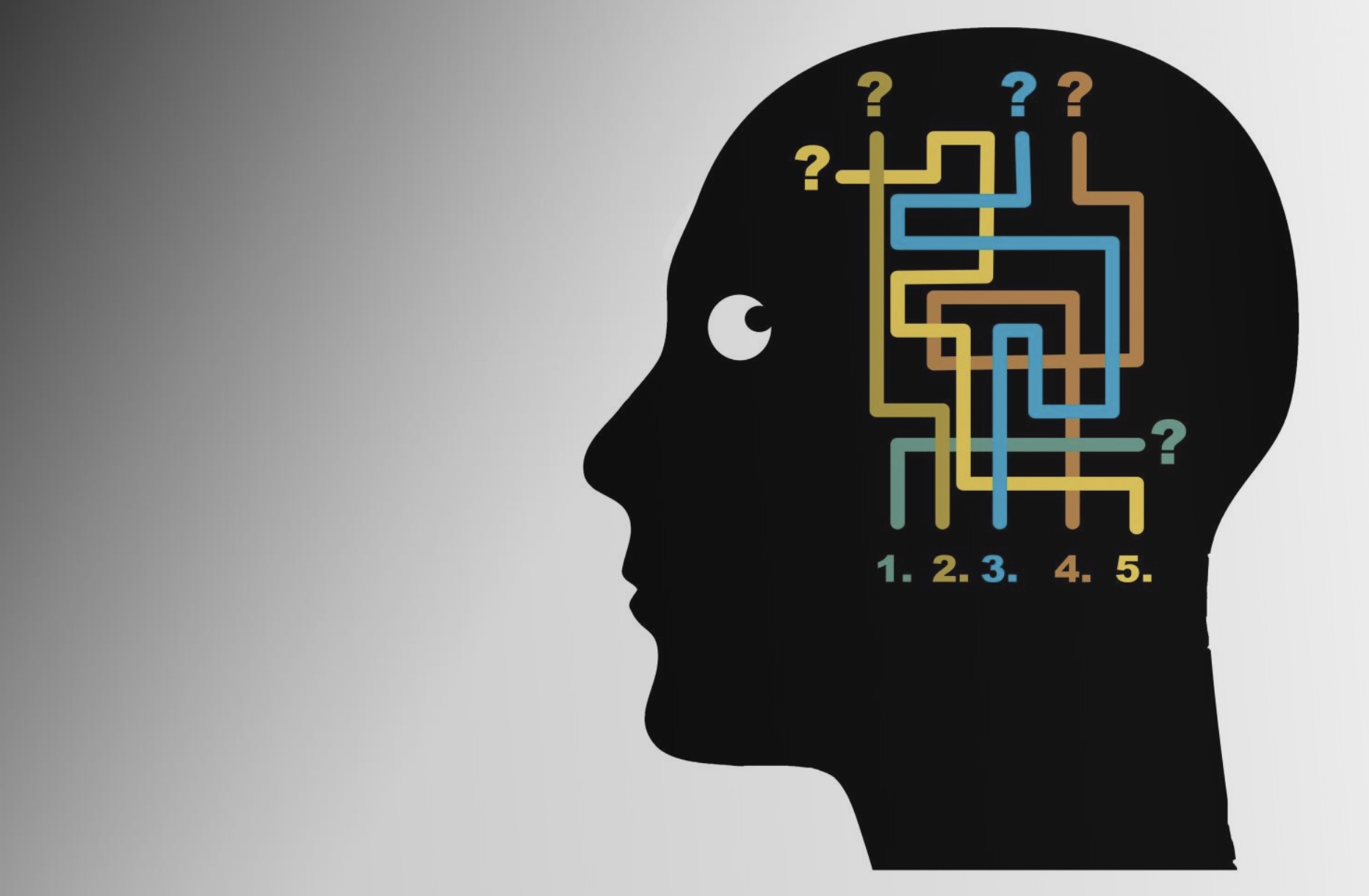
How brain circuits integrate many sources of context to flexibly guide behavior
Picower Institute
September 29, 2021
Mating is instinctual for a mouse but sometimes, for instance when his potential partner smells sick, a male mouse will keep away. When Mark Hyman Jr. Career Development Associate Professor Gloria Choi and colleagues published a study in Nature in April revealing how this primal form of social distancing occurs, they provided an exquisite (and timely) example of how brain circuits factor context into behaviors, making them adaptive and appropriate even when they are innate, or “hardwired.”
When the odor of illness enters the mouse’s nose, that stimulates neurons in its vomeronasal organ to send an electrical signal through a nerve to the brain’s olfactory bulb. Cells there, Choi’s team discovered, relay the signal on to neurons in a region called the cortical amygdala that govern the mating instinct. Finally, completing the health-preserving circuit that will inhibit the mating instinct, those neurons pass on the message to brethren in the neighboring medial amygdalar nucleus. In so doing, this sequence feeds a sensory context, the female’s ill odor, into a circuit to override the default context of an internal state, the instinct to mate. The researchers even showed that by artificially stimulating cortical amygdala neurons they could prevent a mouse from mating with a healthy partner and by artificially silencing those same cells they could make a mouse mate with an ill-smelling one.
As you can learn below, the brain has much greater flexibility in how it operates than the electrical circuits that power your house or even the chips that drive your cell phone. But fundamentally it is the routing of electrical signals from neuron to neuron that forms the basis not only for how we behave, but also how we match behavior appropriately to the circumstances we encounter, Choi said.
“The closest component to behaviors and internal states, and changes in those, are still believed to be neurons and circuits,” she said.
Understanding how brain circuits produce behavior is an exciting area of neuroscience research, including in many Picower Institute labs. Their studies are helping to elucidate how the brain’s anatomy is arranged to process information, and how the many dimensions of flexibility that the central nervous system overlays upon that infrastructure can integrate context to guide appropriate behavior. Context, after all, comes from many sources in many forms—from the senses, like scents and sounds and sights; from internal states, like mating drive or hunger or sleepiness; and even from time and place and from what we’ve learned and remember.
So what were you thinking when you did “this” instead of “that”? You were thinking about the context and relying on your brain’s ability to account for it.
Chemical control
The popular “circuit” metaphor makes it easy to think of neurons as merely switches and wires that pass electrical transmissions from one point to another. And indeed they do that, although instead of being screwed and soldered to metal contacts, they use molecules called neurotransmitters to send signals across tiny junctions called synapses. But if that were all that was going on, the brain would be pretty static and it is anything but. Many members of the Institute’s faculty study how learning occurs and memories are formed when the brain changes its synapses to create or edit circuit connections, but none of that is strictly necessary for existing circuits to flexibly control behaviors that we’ve already learned or that are innate. The brain has other ways to flexibly change how it operates. Choi’s team, for instance, found that the behavioral change of inhibiting mating could not occur without the cortical amygdala neurons also sending a chemical, thyrotrophin releasing hormone (TRH), to the medial amygdalar nucleus neurons.
“Since 1986, that wiring diagram has been staring at researchers,” Flavell quipped. “Many of the small circuits embedded in the wiring diagram have been closely studied, while others haven’t. But a key question that we are trying to answer is how does the whole system work. How are these circuits coupled together to give rise to so-called ‘brain states’?”
In several studies Flavell has shown how a small number of neurons encode contexts and then signal that those circumstances are afoot by releasing chemicals called “neuromodulators” to many other neurons, giving rise to a brain state. Just as TRH may be doing in the circuit Choi uncovered, neuromodulators such as serotonin and dopamine, which are also ubiquitous in humans, add an extra dimension of tuning that can change, or “modulate,” how hardwired circuits process information and output behaviors, Flavell said. Neuromodulators can make neurons more or less electrically excitable given the same degree of input, Flavell explained. They can also make transmission at individual synapses more or less effective.
“The physical connections are like a roadmap, but the way that traffic is actually flowing on the road, the way that neurons are coupled to each other, is dynamic and changes with the animal’s context,” Flavell said. Neuromodulators are one way to make that happen.
For instance, in a 2019 paper in Cell, Flavell’s lab showed how a hungry worm knows to slow down and savor a patch of yummy bacteria when it finds one. A single neuron called NSM extends a little tendril called a neurite into the worm’s pharynx. Equipped with bacterial sensors (that turn out to also be present in the human intestine), the neurite detects when the worm has started to ingest and mash up its food. NSM releases serotonin, which finds its way to many of the neurons in worm’s brain that control locomotion. Upon sensing the serotonin, they hit the brakes.
In a more recent study in bioRxiv, the lab takes their investigation of neuromodulators even further. The study characterizes exactly how serotonin release from NSM modulates that activity of specific neurons in the C. elegans brain. In addition, Flavell’s group found that a neuron called AIA integrates information from sensory neurons about the smell of food. NSM can help determine what it does with that information, depending on whether it detects that the worm is eating or not. If it is, the smell of food (detected by AIA) reinforces that it should stick around to continue dining, a state maintained with serotonin. If the worm isn’t eating, the food smells signal that the animal should go exploring to find the source of that enticing odor. AIA, in that case, can instead trigger neurons that produce a different neuromodulator, called PDF, that cause the worm to start roaming (toward the food odor). Even in the simple circuitry of C. elegans, context changes how neurons interact, giving the animal flexibility to process sensory information.
That neurons capable of emitting neuromodulators can exert far-flung influence over behavior is illustrated by research in Newton Professor Mriganka Sur’s lab, too. There Sur’s team has a focus on a deeply situated, tiny brain region called the locus coeruleus (LC) that happens to supply most of the brain’s norepinephrine. Classically, neuroscientists have regarded norepinephrine from the LC as increasing the brain’s internal state of general arousal, but recent research in the Sur lab suggests it has profound, context-dependent effects on learning and behavior.
For instance, members of the lab have trained mice to expect a reward if they push a lever after hearing a high-pitched tone; the mice also receive an unexpected and irritating puff of air if they mistakenly press the lever after a low-pitched tone. By varying the loudness of the tones, the researchers can also vary the certainty the mice have about what tone they heard. Sur’s lab has found that the louder a high-pitched tone, the more norepinephrine a mouse will send to the motor cortex, which plans movement, before pushing the lever – as if greater certainty prompts it more strongly to push the lever.
Once the lever has been pushed and the mouse gets its feedback of reward or air puff, LC neurons producing norepinephrine then act to fine-tune learning by calling attention to any surprising feedback, Sur’s team has seen. For instance, if the tone was high pitched and faint, but the mouse took the risk to push the lever, the neurons will send a burst of norepinephrine to the prefrontal cortex to note that pleasant surprise. The biggest post-push surge of the neuromodulator, however, occurs when the mouse guesses wrong: that norepinephrine release to the prefrontal cortex appears to signal that the adverse result must be noted. Sure enough, Sur said, the team has seen that the mouse’s performance typically improves after making an error. The LC’s neuromodulatory actions may contribute to that behavioral improvement, though more research is needed to prove it.
Sur’s is not the only research in The Picower Institute showing that the LC communicates with the prefrontal cortex to improve task performance, though. Last November in the Proceedings of the National Academy of Sciences, Picower Professor Susumu Tonegawa’s lab showed that LC norepinephrine neurons connect via distinct circuits to two different parts of the prefrontal cortex to endow mice with both the ability to curb impulses (i.e. to not “jump the gun” when waiting to perform tasks) and to ignore distractions, such as false cues.
Rhythms among regions
Much as the Sur and Tonegawa labs have been investigating the LC, Fairchild Professor Matt Wilson’s lab studies how a different region appears to be a key hub for integrating contexts such as location, motion and memories of reward into behaviors such as navigation: the lateral septum (LS). As rats learn to find and return to the location of a reward in a maze, the lab’s extensive measurements of electrical activity among neurons in the LS shows that those cells are taking in and processing crucial contextual input from many other regions. The LS then appears to package that context to help direct the rat’s navigational plans and actions.
Over the past two years, Wilson and former graduate student Hannah Wirtshafter have published papers in Current Biology and in eLife showing that populations of LS neurons distinctively encode place information coming from the hippocampus, reward information coming from the ventral tegmental area and speed and acceleration information coming from the brainstem. The encoding is apparent in changes in the timing and rate at which the neurons “fire,” or electrically activate, in these different contexts. Some LS neurons, for example, become especially active specifically when the rat nears the reward location. In a new article published in Neuroscience and Biobehavioral Reviews in July, Wilson and Wirtshafter combined their observations with those of other labs to propose that the lateral septum packages all this contextual information into an “integrated movement value signal.”
“The lateral septum has a ton of different inputs,” Wirtshafter said. “What could the animal be doing with place-related firing that’s reward modulated and then velocity and acceleration? The answer, we think, based on where the LS outputs to, is that it is sending a signal about the context and whatever reward is part of that context. It includes what movement needs to be done and whether that movement is worth it in that context.”
While there are ample signs in the research that neuromodulators such as dopamine help the LS communicate about contexts like the feeling of reward, the studies also highlight the key role of another mechanism of flexibility: brain rhythms. Also known as brain waves or oscillations, these rhythms arise from the coordinated fluctuation of electrical activity among neurons that are working in concert. They allow neurons in brain regions to broadcast information and neurons in other regions to tune into those broadcasts, so that they can work together to perform a function, Wilson said.
“These brain dynamics ensure that whoever is sending the information and whoever is receiving the information are doing it at the same time,” Wilson said.
In fact, Picower Professor Earl Miller, who has published numerous studies on how brain rhythms guide the flow of information across the many regions of the brain’s cortex, uses much the same kind of traffic analogy in talking about the function of rhythms that Flavell uses when talking about neuromodulators. Much as those chemicals can, oscillations also flexibly direct the flow of information on the network of “roads” that physical circuit connections create. The traffic metaphor perhaps combines well with the broadcasting one: Just like drivers who tune into a radio traffic report can decide to take an alternate route when they hear about an accident ahead, neurons in a brain region may act differently when they tune into new contextual information coming in from another brain region.
Wilson and Wirtshafter’s research, for example, demonstrates that lateral septum neurons tune into the hippocampus’s broadcast of location information via a specific “theta” frequency of brain waves. In particular, movement through a place is represented by the phase (peak or trough) of the theta waves with which neurons spike.
“In the hippocampus, the phase at which a cell fires during theta can communicate information about the current, prospective, or retrospective spatial location,” Wilson and Wirtshafter wrote in their article. “For instance, …firing of individual hippocampus place cells begins on a particular phase of theta rhythm and progressively shifts forward as the animal moves through the place field.”
So maybe you are not a mouse deciding whether to mate or a rat rooting through a maze for a treat, but you are a person who has stayed out late at a friend’s house. Your internal state is that you are tired. You could head out on long drive home to the reward of your clean, warm bed, or you could sleep on your friend’s notably mustier couch and explain it your spouse the next morning. Then you remember from the drive to your friend’s place earlier, that there was an all-night rest stop along the highway where you could get coffee. Whether you decide to take the wheel or your friend’s offer of the couch will come from how a combination of neuromodulators and rhythms route information along circuits through key brain regions to integrate all this context—your internal state of tiredness, the memory of where that rest stop was, and the reward of your bed (or the punishment of an angry spouse who might ask “What were you thinking?”). Your brain gives you all the flexibility you need.