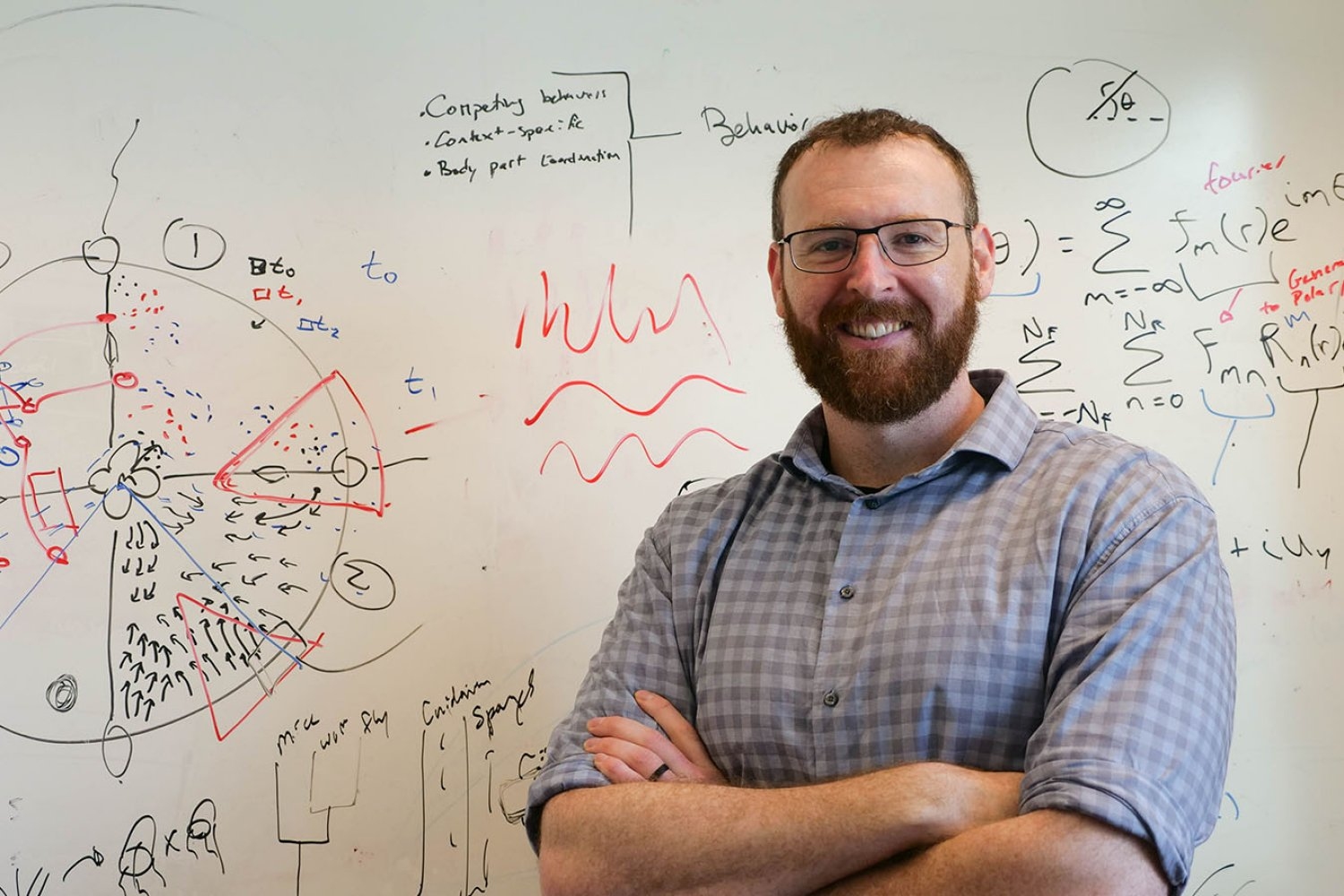
Developing a new neuroscience model is no small feat. New faculty member Brady Weissbourd has risen to the challenge in order to study nervous system evolution, development, regeneration, and function.
Lillian Eden | Department of Biology
May 22, 2023
How does animal behavior emerge from networks of connected neurons? How are these incredible nervous systems and behaviors actually generated by evolution? Are there principles shared by all nervous systems or is evolution constantly innovating? What did the first nervous system look like that gave rise to the incredible diversity of life that we see around us?
Combining the study of animal behavior with studies of nervous system form, function, and evolution, Brandon “Brady” Weissbourd, a new faculty member in the Department of Biology and investigator in The Picower Institute for Learning and Memory, uses the tiny, transparent jellyfish Clytia hemisphaerica, a new neuroscience model.
Q: In 2021, you developed a new model organism for neuroscience research, the transparent jellyfish Clytia hemisphaerica. How do these jellyfish answer questions about neuroscience, the nervous system, and evolution in ways that other models cannot?
A: First, I believe in the importance of more broadly understanding the natural world and diversifying the organisms that we deeply study. One reason is to find experimentally tractable organisms to identify generalizable biological principles — for example, we understand the basis of how neurons “fire” from studies of the squid giant axon. Another reason is that transformative breakthroughs have come from identifying evolutionary innovations that already exist in nature — for example, green fluorescent protein (GFP, from jellyfish) or CRISPR (from bacteria). In both ways, this jellyfish is a valuable complement to existing models.
I have always been interested in the intersection of two types of problems: how nervous systems generate our behaviors; and how these incredible systems were actually created by evolution.
On the systems neuroscience side, ever since working on the serotonin system during my PhD I have been fascinated by the problem of how animals control all of their behaviors simultaneously in a flexible and context-dependent manner, and how behavioral choices depend not just on incoming stimuli but on how those stimuli interact with constantly changing states of the nervous system and body. These are extremely complex and difficult problems, with the particular challenge of interactions across scales, from chemical signaling and dynamic cell biology to neural networks and behavior.
To address these questions, I wanted to move into a model organism with exceptional experimental tractability.
There have been exciting breakthroughs in imaging techniques for neuroscience, including these incredible ways in which we can actually watch and manipulate neuronal activity in a living animal. So, the first thing I wanted was a small and transparent organism that would allow for this kind of optical approach. These jellyfish are a few millimeters in diameter and perfectly transparent, with interesting behaviors but relatively compact nervous systems. They have thousands of neurons where we have billions, which also puts them at a nice intermediate complexity compared to other transparent models that are widely used — for example, C. elegans have 302 neurons and larval zebrafish have something like 100,000 in the brain alone. These features will allow us to look at the activity of the whole nervous system in behaving animals to try to understand how that activity gives rise to behaviors and how that activity itself arises from networks of neurons.
On the evolution side of our work, we are interested in the origins of nervous systems, what the first nervous systems looked like, and broadly what the options are for how nervous systems are organized and functioning: to what extent there are principles versus interesting and potentially useful innovations, and if there are principles, whether those are optimal or somehow constrained by evolution. Our last common ancestor with jellyfish and their relatives (the cnidarians) was something similar to the first nervous system, so by comparing what we find in cnidarians with work in other models we can make inferences about the origins and early evolution of nervous systems. As we further explore these highly divergent animals, we are also finding exciting evolutionary innovations: specifically, they have incredible capabilities for regenerating their nervous systems. In the future, it will be exciting to better understand how these neural networks are organized to allow for such robustness.
Q: What work is required to develop a new organism as a model, and why did you choose this particular species of jellyfish?
A: If you’re choosing a new animal model, it’s not just about whether it has the right features for the questions you want to ask, but also whether it technically lets you do the right experiments. The model we’re using was first developed by a research group in France, who spent many years doing the really hard work of figuring out how to culture the whole life cycle in the lab, injecting eggs, and developing other key resources. For me, the big question was whether we’d be able to use the genetic tools that I was describing earlier for looking at neural activity. Working closely with collaborators in France, our first step was figuring out how to insert things into the jellyfish genome. If we couldn’t figure that out, I was going to switch back to working with mice. It took us about two years of troubleshooting, but now we can routinely generate genetically modified jellyfish in the lab.
Switching to a new animal model is tough — I have a mouse neuroscience background and joined a postdoc lab that used mice and flies; I was the only person working with jellyfish, but had no experience. One of my goals is now to optimize and simplify this whole process so that when other labs want to start working with jellyfish we have a simple aquaculture platform to get them started, even if they have no experience.
In addition to the fact that these things are tiny and transparent, the main reason that we chose this particular species is because it has an amazing life cycle that makes it an exciting laboratory animal.
They have separate sexes that spawn daily with the fertilized eggs developing into larvae that then metamorphose into polyps. We grow these polyps on microscope slides, where they form colonies that are thought to be immortal. These colonies are then constantly releasing jellyfish, which are all genetically identical “clones” that can be used for experiments. That means that once you create a genetically modified strain, like a transgenic line or a knockout, you can keep it forever as a polyp colony — and since the animals are so small, we can culture them in large numbers in the lab.
There’s still a huge amount of foundational work to do, like characterizing their behavioral repertoire and nervous system organization. It’s shocking how little we know about the basics of jellyfish biology — particularly considering that they kill more people per year than sharks and stingrays combined — and the more we look into it, the more questions there are.
Q: What drew you to a faculty position at MIT?
A: I wanted to be in a department that does fundamental research, is enthusiastic about basic science, is open-minded, and is very diverse in what people work on and think about. My goal is also to be able to ultimately link mechanisms at the molecular and cellular level to organismal behavior, which is something that [the] MIT [Department of] Biology is particularly strong at doing. It’s been an exciting first few months! MIT Biology is such an amazing place to do science and it’s been wonderful how enthusiastic and supportive everyone in the department has been.
I was additionally drawn to MIT by the broader community and have already found it so easy to start collaborations with people in neuroscience, engineering, and math. I’m also thrilled to have recently become a member of The Picower Institute for Learning and Memory, which further enables these collaborations in a way that I believe will be transformational for the work in my lab.
It’s a new lab. It’s a new organism. There isn’t a huge, well-established field that is taking these approaches. There’s so much we don’t know, and so much that we have to establish from scratch. My goal is for my lab to have a sense of adventure and fun, and I’m really excited to be doing that here in MIT Biology.