Research Area: Genetics
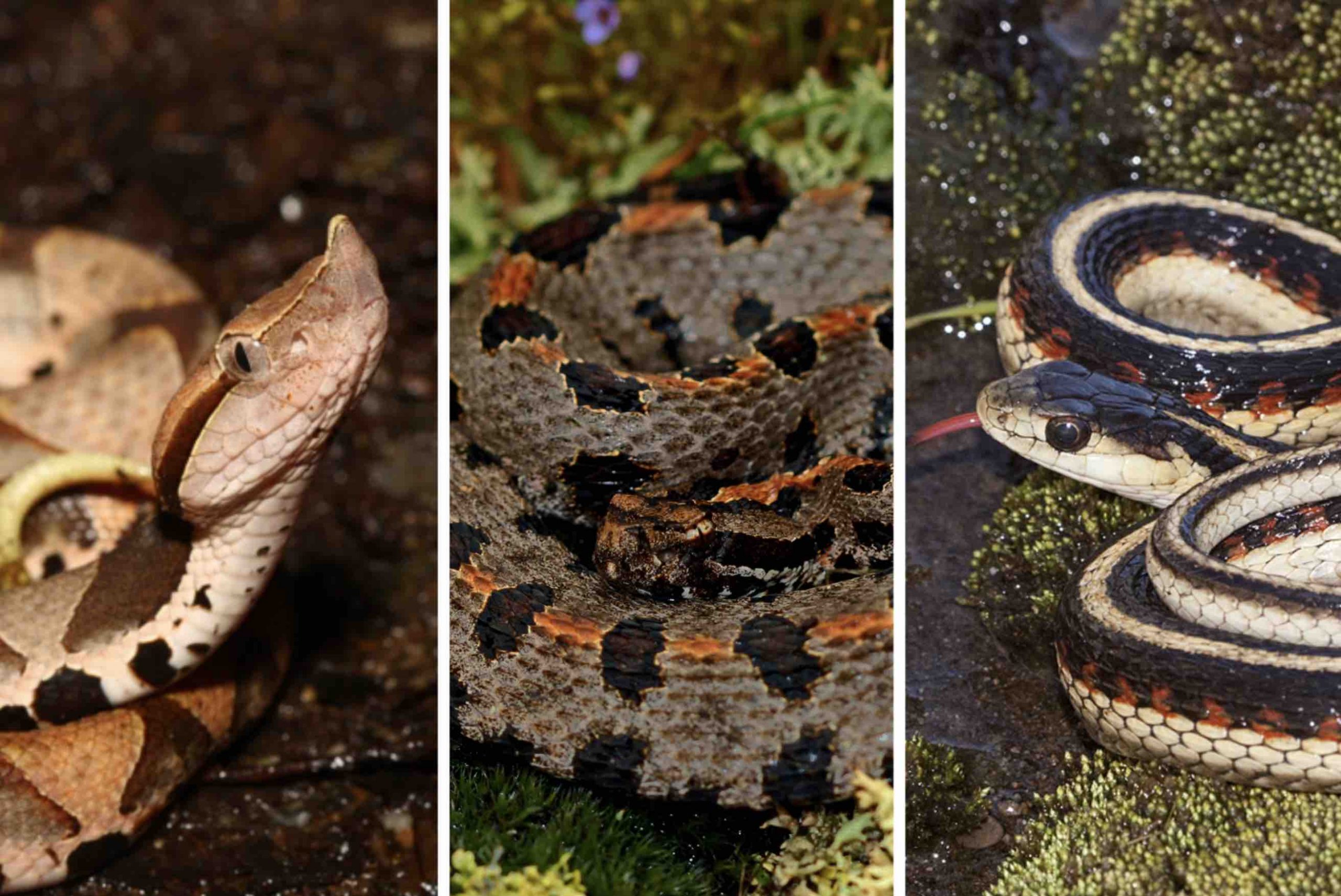
Eva Frederick | Whitehead Institute
January 21, 2021
Sex-specific chromosomes are a dangerous place to be, if you’re a gene. Because these chromosomes — Y chromosomes, in humans — do not have a matching chromosome with which to exchange genetic information, they are prone to losing non-essential genes left and right in a process called genetic decay.
Now, a new study from research scientist Daniel Winston Bellott in the lab of Whitehead Institute Member David Page broadens our understanding of what makes a gene able to survive on a sex-specific chromosome by looking at one especially slithery branch of the evolutionary tree: snakes.
Comparing surviving genes on snake sex-specific chromosomes to those that are lost to the ravages of time can teach scientists about the evolutionary pressures that shaped sex chromosomes as we know them today. “You might think, ‘These are sex chromosomes, so the surviving genes should have something to do with sex, right?’” Bellott said. “But they don’t.”
Instead, many of these genes are essential to the survival of the animal, and take part in key developmental processes. “It turns out that these survivor genes on sex-specific chromosomes may play a very big role in governing how all of the genes across all the chromosomes are read, interpreted and expressed,” said Page, who is also a professor of biology at Massachusetts Institute of Technology (MIT) and an Investigator of the Howard Hughes Medical Institute. “Winston’s study is absolutely foundational to our understanding of what the sex chromosomes are, how the two sexes come to be, and how health and disease traits play out similarly or differently in males and females.”
What is a sex chromosome, anyway?
Over the course of evolution, all sex chromosomes start out as regular, matching chromosomes called autosomes. Then, somewhere along the line, a mutation happens, and one of the chromosomes gains a “switch,” that, when present, causes an embryo to to develop as a specific sex. “It’s actually really easy to make a sex chromosome,” said Bellott. “In most cases, you only need to change one or two genes and you’ve started the sex chromosome system.”
This process has happened numerous times during the course of evolution. It makes sense; sexual reproduction is an efficient way to ensure genetic diversity. But the whole thing is a bit mysterious; are, for example, certain chromosomes predisposed to become sex chromosomes?
That’s where Bellott thought snakes could be especially helpful. “Snakes have a relatively old system of sex chromosomes, where you have a lot of time for the chromosomes to diverge,” Bellott said. “Time has swept away all the genes that aren’t important, and you can see what kind of genes are left.”
Their sex chromosome system also evolved from different autosomes, some 100 million years after humans’, and thus would provide a useful vantage point from which to consider our own genomes.
To learn more about the evolution of these chromosomes, Bellott and Page first gathered a list of “ancestral genes,” which were likely on the chromosome from which the snake sex chromosomes evolved. New sequencing data for several species of animals distantly related to snakes meant that they had a more complete list of these genes — 1,648 to be exact.
Bellott began painstakingly sifting through the genes that remained on the sex-specific chromosomes of three species of snake: the pygmy rattlesnake, mountain garter snake, and the five-pacer viper. He eventually identified 103 ancestral genes that had survived as long as 90 million years of evolution on the snakes’ sex chromosomes. With this list in hand, Bellott could then ask what these surviving genes had in common that set them apart from the hundreds of genes that were swept off the snakes’ sex chromosomes by genetic decay.
What makes a survivor?
To Bellott’s surprise, the genes that remained on the snakes’ sex-specific chromosome had nothing to do with sex determination; neither were they expressed more often in sex-specific tissues, or more often in one sex than the other.
Instead, Bellott and Page’s research identified three key properties that led to a gene’s survival on snake sex-specific chromosomes. First, the gene must be dosage sensitive. In other words, the snake’s body depends on its cells to produce an exact amount of that gene’s protein product. Any more, or any less, and the snake experiences illness or death. Second, a surviving gene is likely broadly expressed in different tissues across the body, not localized to one specific organ or area. And third, surviving genes are subject to strong purifying, or negative, selection. Simply put, this means that if something goes wrong with one of these genes, the snake has a slim chance of survival or producing offspring.
When Bellott dove deeper into the genes’ function, he discovered that for many of them the equivalent gene in humans played a role in key developmental processes such as the formation of the face. When these genes were mutated in humans, their faces — and other essential parts of the body — would not develop properly. “What Winston is seeing here is that the genes that were preserved on the sex specific chromosomes in snakes are disproportionately involved in birth defects in people,” Page said. “We think that nature is selecting for the survival of [sex chromosome] genes whose dosage in certain parts of embryonic development is especially critical.”
In time, Bellott said, this may allow scientists to predict genes whose role in developmental disorders is yet to be discovered. “In some sense, you get to the place where you’re starting to work the experiment backwards in your mind, and say, ‘Let’s take the set of genes that are on sex specific chromosomes in snakes and birds, but that have not yet been implicated in birth defects in humans,’” Page said. “They might be prime candidates to be responsible for heretofore unexplained birth defects.”
From snakes to humans
Next, the researchers sought to broaden their scope. They compared ancestral genes across the three species of snakes and 38 species of birds and mammals with a larger pool of genes that made it to the present day. Many of the surviving genes on bird and mammal chromosomes had different functions than those on snake chromosomes, but again, most had little to do with sex determination.
“Adding the snakes in with the birds and the mammals gave Winston enough data points to be able to see further and to see more precisely, and now for the first time, he was able to confirm something that we had been suspecting for a long time but really didn’t have sufficient data to pin down,” Page said. “And that is that the chromosomes that became sex chromosomes were not sort of inclined to function in sex differences. Before they got picked out of the crowd, they weren’t specialized towards differentiating between the sexes in much of any way.”
Then, as genes were lost over time, evolutionary pressures ensured that the same sort of genes survived. This idea that sex chromosomes — besides their key developmental switch — have little do do with sex determination challenges the common notion of what a sex chromosome actually is.
“I hope people will pick up on this idea that the chromosomes that became sex chromosomes weren’t in any way preordained,” Page said. “They were just ordinary chromosomes out for a walk in the park, and something happened.”
In the future, Bellott and Page plan to further broaden their scope to include other animals, toward the ultimate goal of understanding our own sex chromosomes. “We take these results, and we turn them into a lens through which we look at sex differences in health and disease in our own species,” Page said. “This research really refines our ideas about what it means to be a gene on the human X or Y chromosome, and how we should think about those genes that survive.”
Bellott, D.W. and Page, D.C. “Dosage-sensitive functions in embryonic development drove the survival of genes on sex-specific chromosomes in snakes, birds, and mammals.” Genome Research, Jan. 21, 2021. DOI: 10.1101/gr.268516.120.
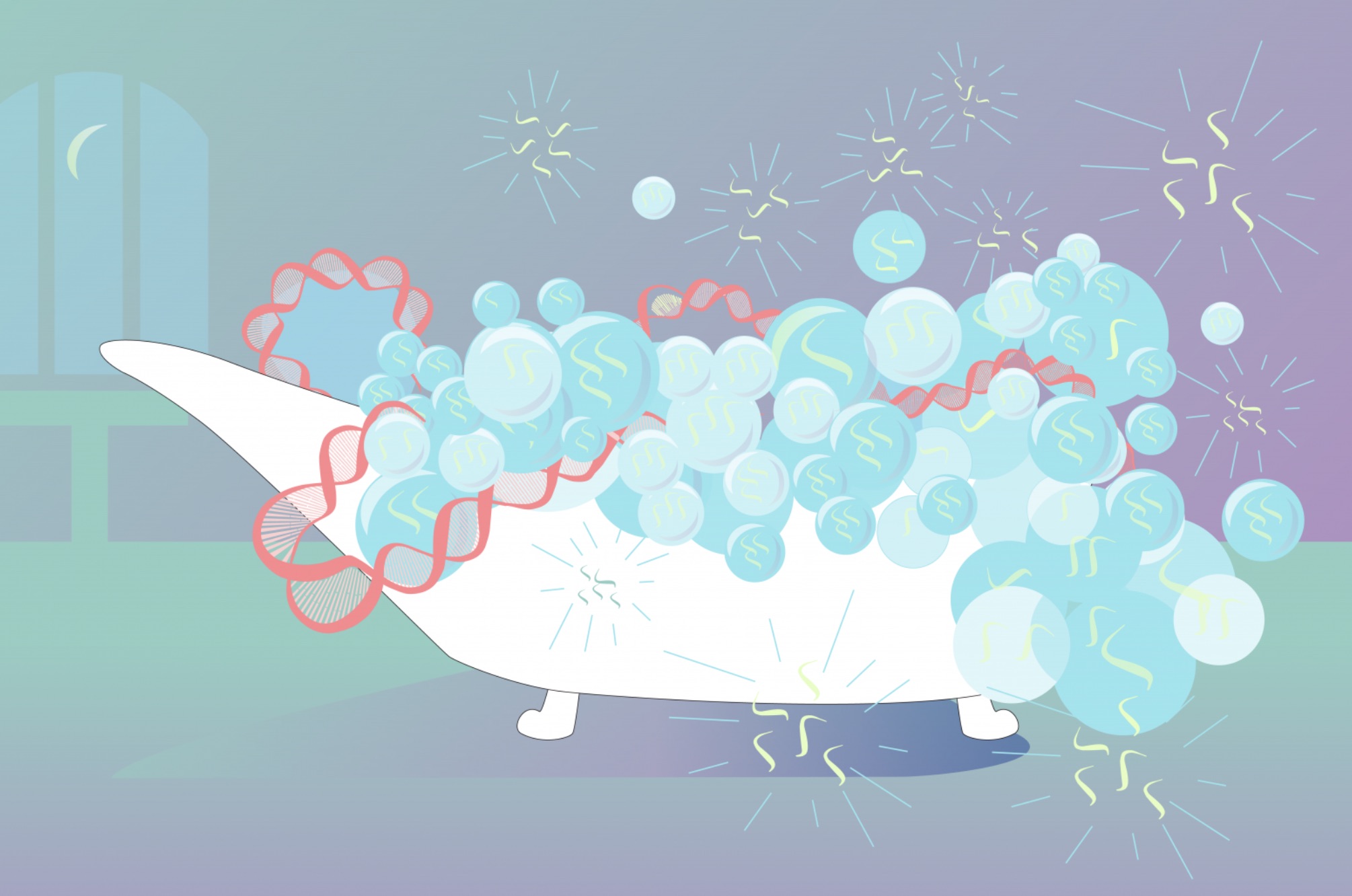
Eva Frederick | Whitehead Institute
December 16, 2020
At any given moment in the human body, in about 30 trillion cells, DNA is being “read” into molecules of messenger RNA, the intermediary step between DNA and proteins, in a process called transcription.
Scientists have a pretty good idea of how transcription gets started: proteins called RNA polymerases are recruited to specific regions of the DNA molecules and begin skimming their way down the strand, synthesizing mRNA molecules as they go. But part of this process is less well understood: how does the cell know when to stop transcribing?
Now, new work from the labs of Whitehead Institute Member Richard Young, also a professor of biology at Massachusetts Institute of Technology (MIT), and Arup K. Chakraborty, professor of chemical engineering, physics and chemistry at MIT, suggests that RNA molecules themselves are responsible for regulating their formation through a feedback loop. Too few RNA molecules, and the cell initiates transcription to create more. Then, at a certain threshold, too many RNA molecules cause transcription to draw to a halt.
The research, published in Cell on December 16, represents a collaboration between biologists and physicists, and provides some insight into the potential roles of the thousands of RNAs that are not translated into any proteins, called noncoding RNAs, which are common in mammals and have mystified scientists for decades.
A question of condensates
Previous work in Young’s lab has focused on transcriptional condensates, small cellular droplets that bring together the molecules needed to transcribe DNA to RNA. Scientists in the lab discovered the transcriptional droplets in 2018, noticing that they typically formed when transcription began and dissolved a few seconds or minutes later when the process was finished.
The researchers wondered if the force that governed the dissolution of the transcriptional condensates could be related to the chemical properties of the RNA they produced — specifically, its highly negative charge. If this were the case, it would be the latest example of cellular processes being regulated via a feedback mechanism — an elegant, efficient system used in the cell to control biological functions such as red blood cell production and DNA repair.
As an initial test, the researchers used an in vitro experiment to test whether the amount of RNA had an effect on condensate formation. They found that within the range of physiological levels observed in cells, low levels of RNA encouraged droplet formation and high levels of RNA discouraged it.
Thinking outside the biology box
With these results in mind, Young Lab postdocs and co-first authors Ozgur Oksuz and Jon Henninger teamed up with physicist and co-first author Krishna Shrinivas, a graduate student in Arup Chakraborty’s lab, to investigate what physical forces were at play.
Shrinivas proposed that the team build a computational model to study the physical and chemical interactions between actively transcribed RNA and condensates formed by transcriptional proteins. The goal of the model was not to simply reproduce existing results, but to create a platform with which to test a variety of situations.
“The way most people study these kinds of problems is to take mixtures of molecules in a test tube, shake it and see what happens,” Shrinivas said. “That is as far away from what happens in a cell as one can imagine. Our thought was, ‘Can we try to study this problem in its biological context, which is this out-of-equilibrium, complex process?’”
Studying the problem from a physics perspective allowed the researchers to take a step back from traditional biology methods. “As a biologist, it’s difficult to come up with new hypotheses, new approaches to understanding how things work from available data,” Henninger said. “You can do screens, you can identify new players, new proteins, new RNAs that may be involved in a process, but you’re still limited by our classical understanding of how all these things interact. Whereas when talking with a physicist, you’re in this theoretical space extending beyond what the data can currently give you. Physicists love to think about how something would behave, given certain parameters.”
Once the model was complete, the researchers could ask it questions about situations that may arise in cells — for instance, what happens to condensates when RNAs of different lengths are produced at different rates as time ensues? — and then follow it up with an experiment at the lab bench. “We ended up with a very nice convergence of model and experiment,” Henninger said. “To me, it’s like the model helps distill the simplest features of this type of system, and then you can do more predictive experiments in cells to see if it fits that model.”
The charge is in charge
Through a series of modeling and experiments at the lab bench, the researchers were able to confirm their hypothesis that the effect of RNA on transcription is due to RNAs molecules’ highly negative charge. Furthermore, it was predicted that initial low levels of RNA enhance and subsequent higher levels dissolve condensates formed by transcriptional proteins. Because the charge is carried by the RNAs’ phosphate backbone, the effective charge of a given RNA molecule is directly proportional to its length.
In order to test this finding in a living cell, the researchers engineered mouse embryonic stem cells to have glowing condensates, then treated them with a chemical to disrupt the elongation phase of transcription. Consistent with the model’s predictions, the resulting dearth of condensate-dissolving RNA molecules increased the size and lifetime of condensates in the cell. Conversely, when the researchers engineered cells to induce the production of extra RNAs, transcriptional condensates at these sites dissolved. “These results highlight the importance of understanding how non-equilibrium feedback mechanisms regulate the functions of the biomolecular condensates present in cells,” said Chakraborty.
Confirmation of this feedback mechanism might help answer a long-standing mystery of the mammalian genome: the purpose of non-coding RNAs, which make up a large portion of genetic material. “While we know a lot about how proteins work, there are tens of thousands of noncoding RNA species, and we don’t know the functions of most of these molecules,” said Young. “The finding that RNA molecules can regulate transcriptional condensates makes us wonder if many of the noncoding species just function locally to tune gene expression throughout the genome. Then this giant mystery of what all these RNAs do has a potential solution.”
The researchers are optimistic that understanding this new role for RNA in the cell could inform therapies for a wide range of diseases. “Some diseases are actually caused by increased or decreased expression of a single gene,” said Oksuz, a co-first author. “We now know that if you modulate the levels of RNA, you have a predictable effect on condensates. So you could hypothetically tune up or down the expression of a disease gene to restore the expression — and possibly restore the phenotype — that you want, in order to treat a disease.”
Young added that a deeper understanding of RNA behavior could inform therapeutics more generally. In the last 10 years, a variety of drugs have been developed that directly target RNA successfully. “RNA is an important target,” Young said. “Understanding mechanistically how RNA molecules regulate gene expression bridges the gap between gene dysregulation in disease and new therapeutic approaches that target RNA.”
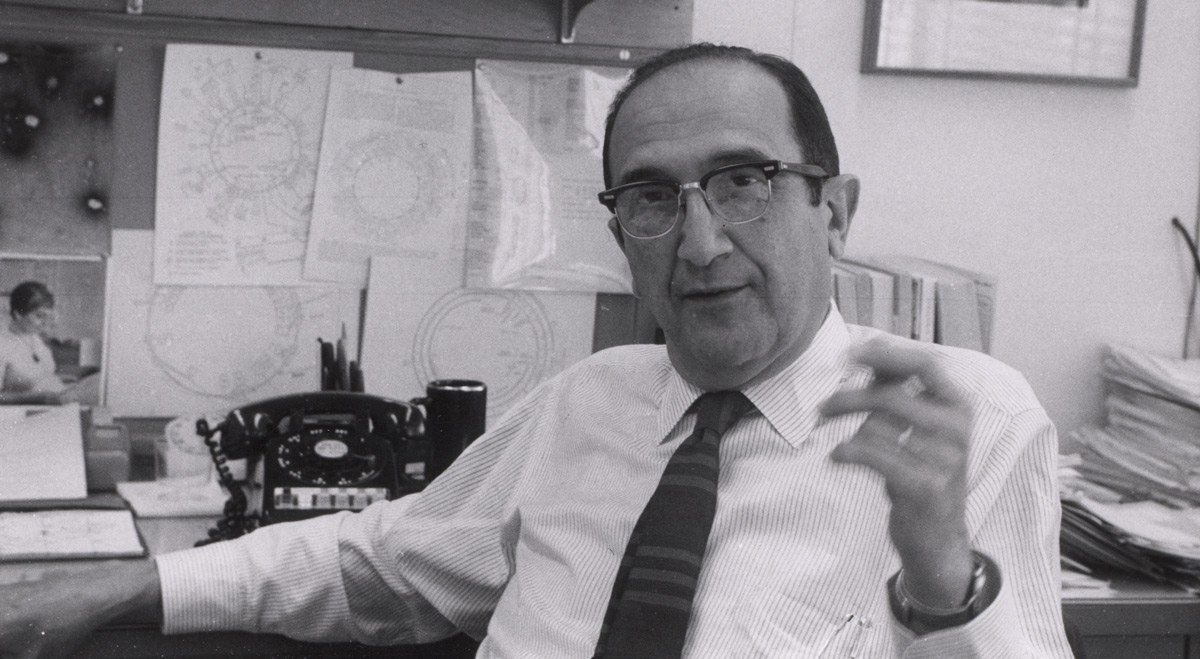
Salvador Luria is known for his research on phage genetics, but his lab’s contribution to the discovery of restriction enzymes also sparked important technological advances.
Saima Sidik
December 15, 2020
In the early 1950s, a woman named Mary Human found the first evidence of a group of proteins called restriction enzymes — a discovery that would reverberate throughout the research community for decades. But many important discoveries, from penicillin to medical X-rays, are inspired by a messy fluke rather than carefully reasoned logic, and Human’s discovery was no different.
Fortunately, Human’s boss was a jovial scientist named Salvador Luria, who appreciated that life’s quirks often yield the most valuable results — so much so that he wrote a 1955 Scientific American article in which he praised Human’s approach. “It often pays to do somewhat untidy experiments, provided one is aware of the element of untidiness,” he wrote.
Indeed, Luria’s life was far from being a tidy package. This Italian native fled Europe to escape Nazis, was briefly blacklisted by the NIH presumably because of his vocal opposition to American foreign policy, and suffered from depression despite his outwardly cheery appearance. But Luria’s life was also extraordinary. He earned a medical degree in Torino, Italy, but decided he preferred performing research over practicing medicine. After leaving Europe in the 1940s to escape the persecution of Jews like himself, he held professorships at three American institutions, including MIT. He was known as an insightful scientist, a kind colleague, and a thoughtful mentor, right up until his death in 1991.
A Surprising Observation in the Midwest
For much of his career, Luria applied his keen insight to phages — viruses that invade and kill bacteria. He and two collaborators won the Nobel Prize after realizing that genetic mutations in bacteria can protect them from deadly phages. But the untidy experiment Luria referred to in his Scientific American article related to a lesser-known aspect of his lab’s phage work: restriction enzymes, which cut DNA at specific places. Luria was the first person to find evidence of these critical tools, which opened a whole new field of genetic manipulation. A cascade of research spanning two decades eventually led a scientist supervised by Luria’s former research associate to win a Nobel prize for characterizing these enzymes, which catalyzed modern molecular biology.
The restriction enzyme story starts in the late 1940s, when Luria was a professor at Indiana University. He noticed that a phage called T2 didn’t seem to grow inside and kill certain mutant strains of Escherichia coli. T2 always killed the first batch of mutant E. coli, but when he tested whether a new batch of the same type of bacteria would catch the virus from the dead bacteria, the new batch didn’t succumb to the virus.
In 1950, Luria moved to the University of Illinois, Urbana, where one of his employees, a woman named Mary Human, continued to work on the T2 mystery. One day, in the midst of an experiment, Human realized she’d run out of the strain of E. coli she usually used, and this is where the experiment got a little untidy. Instead of waiting to do the experiment on another day with a healthy batch of E. coli, Human mixed phage-killed E. coli with a different type of bacteria called Shigella. T2 always seemed to act the same in Shigella as it did in E. coli, so she didn’t expect the switch to matter. But the next morning, the Shigella were dead! It seemed that T2 could only reproduce once in the particular mutant strain of E. coli that Human was studying, but when she moved T2 from these mutant E. coli to Shigella, it restored the virus’ ability to reproduce. Human and Luria concluded that something about the mutant E. coli changed the T2, and limited the kinds of bacteria in which it could grow.
At the time, Human and Luria couldn’t explain what was happening to T2 in these mutant bacteria. Luria went about his career, still carrying this mystery with him.
An explanation in Cambridge, Massachusetts
In 1958, Luria came to MIT Biology for a sabbatical. The structure of DNA had been discovered just five years earlier, and MIT needed someone who understood its implications to usher the Institute into the genomics era. Luria was renowned for his ability to predict which direction biology would move, so the Institute wanted him to fill this role. At the end of his sabbatical, Luria accepted a permanent position in MIT Biology, where he stayed for the rest of his career.
“I asked Luria if he thought it was possible to do molecular biology with animal viruses, and he said, ‘I don’t know, why don’t you find out and tell me?’” Baltimore says.In addition to being a skilled scientist, Luria was a thoughtful mentor. David Baltimore, professor at the California Institute of Technology, was one of Luria’s early mentees at MIT. At the time, most research into viruses focused on the phages that Luria studied, but Baltimore wanted to break new ground by studying viruses that infect animals. He credits Luria for encouraging him to go down this path — one that led him to become a Nobel Laureate himself.
In addition to being a skilled scientist, Luria was deeply opposed to McCarthyism and the Vietnam War, and he devoted a lot of time to political activism like writing letters, to newspaper editors as well as to other scientists, trying to gather support for his views.
Fortunately, Luria had a deputy to help him run his lab while he was revamping MIT Biology and trying to stop the war. “If you wanted to know something on a daily basis, you went to Helen Revel,” recalls Costa Georgopoulos, a professor at the University of Utah who earned his PhD in Luria’s lab in the 1960s.
Revel earned her PhD with MIT Biology’s Boris Magasanik before becoming Luria’s research associate. “Those days, women were not readily made professors, so she worked on Luria’s grants,” Georgopoulos says.
Georgopoulos describes Revel as reserved and meticulous. She didn’t advertise her skill as a scientist; she just got to work. With this attitude, she led the scientists who figured out the mystery of the mutant bacteria that changed the T2 phage.
Since Human’s fortuitously messy experiment, a lineage of phage researchers that originated in Luria’s lab had learned a lot about how bacteria and phages interact. First, Luria’s former research associate, Guiseppe Bertani, showed that phages other than T2 also behave differently in different types of bacteria. Later, Bertani’s own research associate, Werner Arber, went on to discover that bacteria can mark the DNA of phages that replicate within them. When marked phages try to enter new bacteria, the marks can signal that the phages are foreign invaders, allowing the new bacteria to kill the phages. Arber and two of his colleagues, Daniel Nathans and Hamilton O. Smith, eventually won their own Nobel prize for their work on restriction enzymes.
Certain bacteria mark phage DNA by replacing one of the bases that make up the genetic code, called cytosine, with a modified version called 5-hydroxymethylcytosine. Revel, with help from Luria, Georgopoulos, and others, found that the T2 phage takes this system one step farther by using a bacterial enzyme to attach sugars to modified cytosines. Some mutant bacteria are unable to transfer sugars to phage cytosines, and so the phages grown in these bacteria come out “sour” instead of “sweet,” as Luria wrote. Restriction enzymes recognize these sweet-natured phages as foreign, and destroy them.
As researchers learned more about restriction enzymes, they realized that they can work in all sorts of ways. Bacteria can also mark their own DNA to prevent restriction enzymes from cutting it, allowing certain kinds of restriction enzymes to cut naked DNA sequences in the genomes of invading phages. Soon, biologists realized that restriction enzymes would let them cut any kind of DNA, not just phage genomes. This discovery had many consequences, one of which was that scientists could paste snipped DNA back together in new combinations. Many people were initially wary that combining DNA from different organisms could have unintended consequences. But by the 1980s, scientists had harnessed restriction enzymes for a whole host of safe purposes, and technologies centered around these enzymes continue to evolve.
Today, after decades of work, scientists have used restriction enzymes to study genetic variations in humans, find sequences that cause disease, identify relationships between people, and solve crimes. Scientists have used restriction enzymes to make proteins glow like jellyfish, to study the structure of DNA, and to make bacteria produce insulin.
T2 phages and their relationship to restriction enzymes are just one area of biology where Luria and his lab made profound contributions. Among his biggest achievements was recruiting and employing many forward-thinking scientists who built MIT Biology into the department it is today. In fact, as the first director of the Center for Cancer Research, Luria recruited Phillip Sharp, who would go on to win a Nobel Prize for discovering RNA splicing. Sharp joined a center that already included David Baltimore, as well as current MIT Biology professors Nancy Hopkins and Robert Weinberg, all of whom have made huge contributions to cancer research.
Scientists had just begun to elucidate the link between genetics, viruses, and cancer in the early 1970s, but Baltimore says that Luria was often the first person to jump on new applications for the techniques and thinking underlying molecular biology.
“Luria’s genius was understanding where biology was going,” says Baltimore. “At every stage, he was wondering what the next step would be.” But even geniuses need a messy fluke like Human’s now and then.
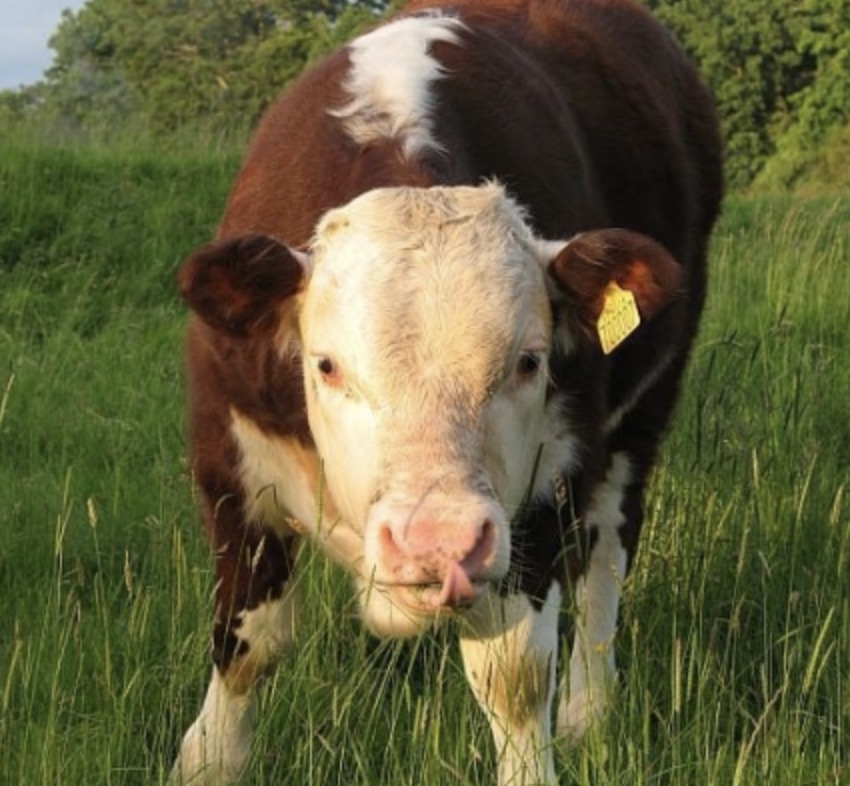
Whitehead Institute
November 18, 2020
In a new study, published Nov. 18 in the journal Genome Research, scientists in the lab of Whitehead Institute Member David Page present the first ever full, high-resolution sequence of the Y chromosome of a Hereford bull. The research, more than a decade in the making, suggests that bulls’ Y chromosomes have evolved dozens of copies of the same genes in a selfish attempt to make more males — a move that is countered in the female-determining X chromosome.
“When you have an X and a Y chromosome, it’s a setup for conflict,” said Page, who is also a professor of biology at the Massachusetts Institute of Technology and investigator with the Howard Hughes Medical Institute. “Seeing this full blown competition between the cattle X and Y means we have to think more deeply about this conflict as a constant and general feature of sex chromosomes in mammals.”
This insight into the forces that govern sex chromosome behavior and evolution will help scientists in Page’s lab study genetic differences between males and females and how they play out in health and disease across every part of the body, Page added.
Of mice, men and cattle
Sex chromosomes — the X and the Y — evolved from a regular pair of symmetrical chromosomes some 200 million years ago. Those born biologically female have two X chromosomes. Those born biologically male have one X and one Y.
Page’s lab successfully sequenced the human Y chromosome in 2003, and afterwards the researchers wanted to be able to compare the sequence to its counterparts in other animals in order to help understand how they have evolved and diverged over time.
To make these comparisons, researchers in Page’s lab laid out a list of several mammals — including chimps, opossums, and mice — that occupied different branches of the mammalian family tree. One after another, the scientists began sequencing these creatures’ Ys, using a high-resolution sequencing method called SHIMS — short for Single-Haplotype Iterative Mapping and Sequencing — to obtain a level of detail that other techniques, like shotgun sequencing, can’t.
This powerful sequencing technology allowed the researchers to observe a strange peculiarity of Y chromosomes: in some species, nearly all of the genetic material on the Y is made up of sequences of DNA that have been amplified dozens or hundreds of times over — “like a hall of mirrors,” Page said.
In mice, for example, repeats of just a few testis-specific genes make up nearly 98 percent of the Y chromosome. In humans, however, repeats make up only about 45 percent. “We wanted to know if this was just a peculiarity of rodents, or if other Y chromosomes might come close,” Page said.
That’s where the bull came in. “Outside of primates and rodents, the next branch off the mammalian tree includes bull,” said Jennifer Hughes, a researcher in Page’s lab and the first author of the paper. “We didn’t know if the bull’s Y chromosome would look like a mouse Y or a human Y or something else entirely.”
The running of the bull’s (sequencing data)
It took the Page Lab and collaborators at Baylor College of Medicine’s Human Genome Sequencing Center, the McDonnell Genome Institute at Washington University, Texas A&M University, and other institutions more than a decade to tease apart the complexities of the bull Y chromosome. In fact, it turned out to be the most gene-dense of any Y chromosome ever mapped — largely due to the fact that 96 percent of its genetic material was made up of repetitive sequences.
As in the mouse, most of the bull’s “hall of mirrors” repeats appeared to be expressed in the testis. But the question remained: Why? “What drives it can’t just be purely making more sperm, because that’s just overkill, right?” Hughes said. “You don’t really need hundreds of copies of a gene to accomplish that task.”
The researchers found a clue when they took a closer look at the bovine X chromosome: the female-determining sex chromosome also had a few copies of these testis-specific genes. “We don’t really know the mechanism in the bull, but the thought is that somehow the amplification of these genes in the Y has to do with helping the Y get passed on — and the X copies are amplified to compete against that tendency and help the X,” Hughes said.
A selfish pursuit
This X-Y arms race has been proven to happen in mice: somehow, repetitive genes on the Y chromosome give it an extra edge when it comes to ending up in the sperm during gamete formation. In a 2012 study, researchers knocked out the Y-chromosome repeats. Without the extra genes, more X chromosomes than Ys ended up in sperm cells, and the sex ratio of offspring skewed female. Over years of evolution, the X has developed repeats as well — its own way to get a leg up in the race.
Competition between X and Y chromosomes is selfish, Hughes said, because it’s not a good thing for the species to have a skewed sex ratio. Thus, these alterations benefit only the lucky chromosome that ends up in the fertilized egg. The fact that a selfish — and even detrimental — mechanism would continue for millions of years in disparate branches of the evolutionary tree suggests that these conflicts may be an inevitable side effect of having a pair of asymmetrical sex chromosomes. “These X-Y arms races have probably been around for as long as mammals have been around,” Page said.
Evolutionary theory aside, knowing the mechanisms controlling the sex ratios of cattle could be of practical use in the coming years. “It could be of great interest to breeders, because they would love to be able to manipulate the sex of cattle offspring,” Hughes said. “For example, dairy farmers would prefer more females and meat farmers would prefer more males.”
Right now, the lab is working on leafing out the branches of their Y chromosome evolutionary tree. The bull’s is the seventh sex chromosome to be completely sequenced using the SHIMS method. Hughes, Page and the lab are also eyeing members of other animal groups, including reptiles.
“Our lab is focused on sex differences across the human body, and all of that work really is inspired by lessons that we’ve learned by comparing the Y chromosomes of different animals with our own,” Page said. “It’s like when you go to an art gallery and just sit on a bench and look and feel inspired — these sequences are an infinite source of inspiration in the work we are doing. And we can now add the bull to our gallery.”
***
Hughes, J. et al. “Sequence analysis in Bos taurus reveals pervasiveness of X-Y arms races in mammalian lineages.” Genome Research, Nov. 18, 2020. DOI: 10.1101/gr.269902.120
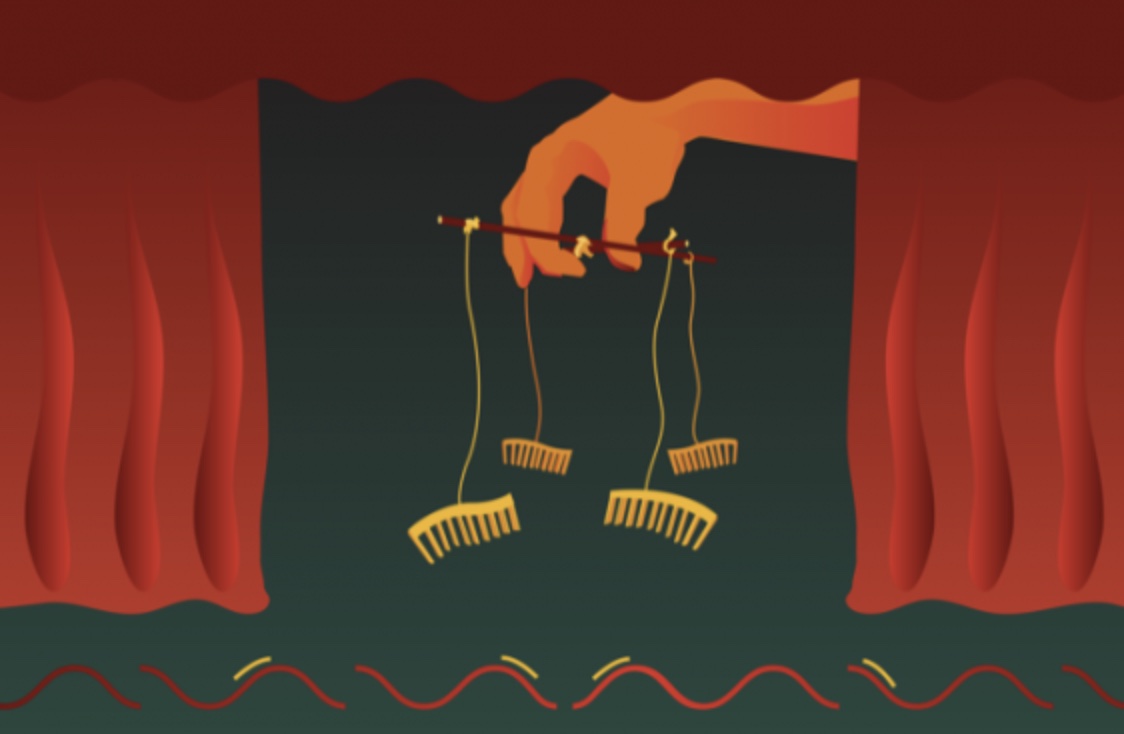
Whitehead Institute
November 12, 2020
MicroRNAs are short RNA sequences that maintain a tight control on which genes are expressed and when. They do this by regulating which messenger RNA (mRNA) transcripts — the single-stranded templates for proteins — are actually read by the cell. But what controls these cellular controllers?
In a new study published Nov. 12 in Science, researchers in David Bartel’s lab at Whitehead Institute show that mRNAs and other RNAs often turn the tables on their microRNA regulators — and show that the path to microRNA degradation is not what scientists expected it to be.
“A lot of people know that microRNAs repress mRNAs — that’s textbook,” said Charlie Shi, a graduate student in Bartel’s lab and first author on the paper. “But in certain cases, this logic is reversed. And I think that’s really interesting and weird, this idea that often the tables are turned.”
When transcripts attack
MicroRNAs typically control gene expression by binding to mRNA transcripts, and then working together with a protein called Argonaute to “silence” those transcripts by causing them to be more rapidly degraded. Because microRNAs are held cozily inside of the Argonaute protein, they are shielded from destructive enzymes in the cell, and are thus fairly long-lived by cellular standards. They can persist for up to a week, causing the destruction of many mRNA molecules over that time.
Sometimes, however, a microRNA binds to a special target site on an mRNA transcript that leads to premature destruction of the microRNA. This phenomenon — called target-directed microRNA degradation, or TDMD — happens naturally in cells, and is a way to control how much of certain microRNAs are allowed to persist at any given time.
Bartel’s lab began studying this form of degradation after researchers in the lab discovered that an RNA called CYRANO, which doesn’t code for any proteins, leads to the degradation of a specific microRNA called miR-7. This interaction was interesting to the researchers because the mechanism did not seem to line up with the current theories about TDMD.
Previous models of TDMD suggested that special target sites, like the one in CYRANO, cause one end of the microRNA to stick out of Argonaute and become vulnerable to the addition and subtraction of nucleotides by cytoplasmic enzymes. This process, called tailing and trimming, was thought to be a key step in the path to degradation of the microRNA.
“But when you knock out the enzyme that causes tailing of miR-7, it has no impact on the degradation,” Shi said. “So that’s curious, right? So how can we really perturb this supposedly responsible system and have no impact?”
A new model
In order to further probe the mechanism of TDMD, the researchers focused in on this interaction between the CYRANO noncoding RNA and miR-7. Shi designed a CRISPR screen to identify genes essential for the microRNA’s degradation when it encountered a CYRANO transcript.
The screen yielded one gene that was essential to the microRNA’s degradation, called ZSWIM8. When they looked up the gene’s function, the researchers found that it codes for a component of a ubiquitin ligase. Ubiquitin — so named because it is found in virtually all types of cells — serves as a flag to mark proteins for degradation in a cellular garbage disposal called the proteasome.
The finding of the ZSWIM8 ubiquitin ligase implied that CYRANO-mediated microRNA degradation involves destruction of the Argonaute protein. In this new molecular model for TDMD, the regulating RNA, CYRANO, binds to the microRNA, mir-7, encased in its protective Argonaute protein, and then recruits the ZSWIM8 ubiquitin ligase. This ligase then sticks a few ubiquitin molecules onto the microRNA’s Argonaute, leading Argonaute to be degraded, and thereby exposing its microRNA cargo to be destroyed by enzymes in the cell. Importantly, this process does not require any trimming and tailing of the microRNA.
“The discovery of this unanticipated pathway for TDMD illustrates the power of CRISPR screens, which can simultaneously query essentially every protein in the cell, including those that you never dreamed would be involved,” said Bartel, who is also an investigator of the Howard Hughes Medical Institute and a professor of biology at Massachusetts Institute of Technology.
A multitude of microRNAs
When the researchers looked at other known examples of TDMD, they found the ZSWIM8 was essential in all of them. Having identified this key part of the degradation pathway allowed them to seek out more microRNAs that are subject to this regulation.
“When we started this project, there were only around four examples in nature of endogenous RNAs that are encoded by the cell that can perform TDMD,” Shi said. “We had a feeling that there would be many more, and so by finding a factor that was required for TDMD in a general way — ZSWIM8 –we were then able to ask and answer the question, ‘how widespread this phenomenon?’”
As it turns out, TDMD is fairly common in multicellular organisms. The researchers looked for evidence of the microRNA degradation mechanism in different cell types — two from mice, and one from fruit flies — and found that in any given cell, up to 20 different microRNAs were regulated by TDMD out of a couple hundred total microRNAs in the cell.
The researchers also observed this mechanism in human cells and nematodes, suggesting that TDMD as a method for regulating microRNAs dates back to the common ancestor of these disparate species. That definitely creates a lot of questions for us,” Shi said. “Each one of these microRNAs is a story.”
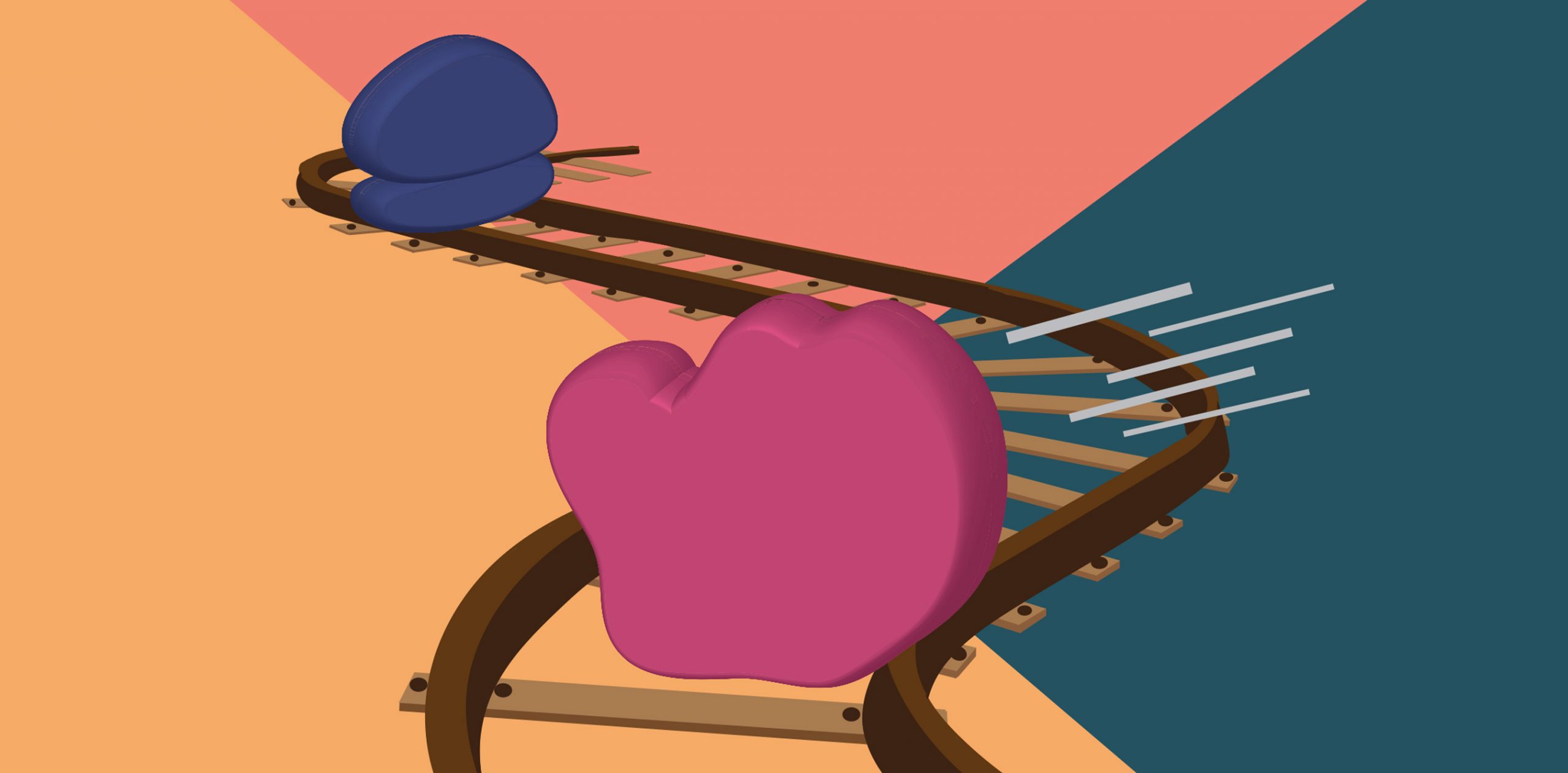
Researchers discover new rules governing bacterial gene expression that overturn fundamental assumptions about basic biological pathways.
Raleigh McElvery
August 26, 2020
On the evolutionary tree, humans diverged from yeast roughly one billion years ago. By comparison, two seemingly similar species of bacteria, Escherichia coli and Bacillus subtilis, have been evolving apart for roughly twice as long. In other words: walking, talking bipeds are closer on the tree of life to single-celled fungus than these two bacteria are to one another. In fact, it’s becoming increasingly clear that what is true of one bacterial type may not be true of another — even when it comes down to life’s most basic biological pathways.
E. coli has served as a model organism in scientific research for over a century, and helped researchers parse many fundamental processes, including gene expression. In these bacteria, as one molecular machine, the RNA polymerase, moves along the DNA transcribing it into RNA, it is followed in close pursuit by a second molecular machine, the ribosome, which translates the RNA into proteins. This “coupled” transcription-translation helps monitor and tune RNA output, and is considered a hallmark of bacteria.
However, an interdisciplinary team of biologists and physicists recently showed that the B. subtilis bacterium employs a different set of rules. Rather than working in tandem with the ribosome, the polymerase in B. subtilis speeds ahead. This system of “runaway” transcription creates alternative rules for RNA quality control, and provides insights into the sheer diversity of bacterial species.
“Generations of researchers, including myself, were taught that coupled transcription-translation is fundamental to bacterial gene expression,” says Gene-Wei Li, an associate professor of biology and senior author of the study. “But our very precise, quantitative measurements have overturned that long-held view, and this study could be just the tip of the iceberg.”
Grace Johnson, a graduate student in the Department of Biology, and Jean-Benoît Lalanne, a graduate student in the Department of Physics, are the lead authors on the paper, which appeared in Nature on Aug. 26.
A curious clue
In 2018, Lalanne developed an experimental technique to measure the boundaries of RNA transcripts. When DNA is transcribed into RNA, the resulting transcripts are generally longer than the DNA coding sequence because they also have to include an extra bit at the end to signal the polymerase to stop. In B. subtilis, Lalanne noticed there simply wasn’t enough space between the ends of the coding sequences and the ends of the RNA transcripts — the extra code was too short for both the polymerase and the ribosome to fit at the same time. In this bacterium, coupled transcription-translation didn’t seem possible.
“It was a pretty weird observation,” Lalanne recalls. “It just didn’t square up with the accepted dogma.”
To delve further into these puzzling observations, Johnson measured the speeds of the RNA polymerase and ribosome in B. subtilis. She was surprised to find that they were moving at very different rates: the polymerase was going roughly twice as fast as the ribosome.
During coupled transcription-translation in E. coli, the ribosome is so closely associated with the RNA polymerase that it can control when transcription terminates. If the RNA encodes a “premature” signal for the polymerase to stop transcribing, the nearby ribosome can mask it and spur the polymerase on. However, if something goes awry and the ribosome is halted too far behind the polymerase, a protein called Rho can intervene to terminate transcription at these premature sites, halting the production of these presumably non-functional transcripts.
However, in B. subtilis, the ribosome is always too far behind the polymerase to exert its masking effect. Instead, Johnson found that Rho recognizes signals encoded in the RNA itself. This allows Rho to prevent production of select RNAs while ensuring it doesn’t suppress all RNAs. However, these specific signals also mean Rho likely has a more limited role in B. subtilis than it does in E. coli.
A family trait
To gauge how common runaway transcription is, Lalanne created algorithms that sifted through genomes from over 1,000 bacterial species to identify the ends of transcripts. In many cases, there was not enough space at the end of the transcripts for both the RNA polymerase and the ribosome to fit, indicating that more than 200 additional bacteria also rely on runaway transcription.
“It was striking to see just how widespread this phenomenon is,” Li says. “It raises the question: How much do we really know about these model organisms we’ve been studying for so many years?”
Carol Gross, a professor in the Department of Microbiology and Immunology at University of California San Francisco who was not involved with the study, refers to the work as a “tour de force.”
“Gene-Wei Li and colleagues show transcription-translation coupling, thought to be a foundational feature of bacterial gene regulation, is not universal,” she says. “Instead, runaway transcription leads to a host of alternative regulatory strategies, thereby opening a new frontier in our study of bacterial strategies to thrive in varied environments.”
As researchers widen their experimental radius to include more types of bacteria, they are learning more about the basic biological processes underlying these microorganisms — with implications for those that take up residence in the human body, from helpful gut microbes to noxious pathogens.
“We’re beginning to realize that bacteria can have distinct ways of regulating gene expression and responding to environmental stress,” Johnson says. “It just shows how much interesting biology is left to uncover as we study increasingly diverse bacteria.”
Citation:
“Functionally uncoupled transcription–translation in Bacillus subtilis”
Nature, online August 26, 2020, DOI: 10.1038/s41586-020-2638-5
Grace E. Johnson, Jean-Benoît Lalanne, Michelle L. Peters, and Gene-Wei Li
Top illustration: Researchers discovered a new system of transcription and translation in bacteria, where the polymerase (pink) in B. subtilis “runs away” from the ribosome (blue). Credit: Grace Johnson
Posted: 8.26.20
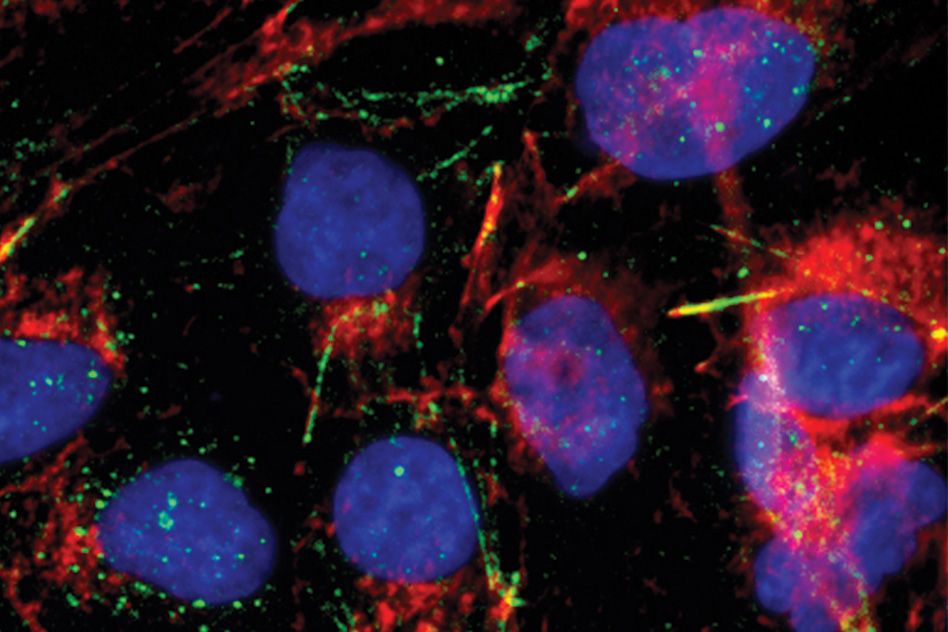
ENCODE consortium identifies RNA sequences that are involved in regulating gene expression.
Anne Trafton | MIT News Office
July 29, 2020
The human genome contains about 20,000 protein-coding genes, but the coding parts of our genes account for only about 2 percent of the entire genome. For the past two decades, scientists have been trying to find out what the other 98 percent is doing.
A research consortium known as ENCODE (Encyclopedia of DNA Elements) has made significant progress toward that goal, identifying many genome locations that bind to regulatory proteins, helping to control which genes get turned on or off. In a new study that is also part of ENCODE, researchers have now identified many additional sites that code for RNA molecules that are likely to influence gene expression.
These RNA sequences do not get translated into proteins, but act in a variety of ways to control how much protein is made from protein-coding genes. The research team, which includes scientists from MIT and several other institutions, made use of RNA-binding proteins to help them locate and assign possible functions to tens of thousands of sequences of the genome.
“This is the first large-scale functional genomic analysis of RNA-binding proteins with multiple different techniques,” says Christopher Burge, an MIT professor of biology. “With the technologies for studying RNA-binding proteins now approaching the level of those that have been available for studying DNA-binding proteins, we hope to bring RNA function more fully into the genomic world.”
Burge is one of the senior authors of the study, along with Xiang-Dong Fu and Gene Yeo of the University of California at San Diego, Eric Lecuyer of the University of Montreal, and Brenton Graveley of UConn Health.
The lead authors of the study, which appears today in Nature, are Peter Freese, a recent MIT PhD recipient in Computational and Systems Biology; Eric Van Nostrand, Gabriel Pratt, and Rui Xiao of UCSD; Xiaofeng Wang of the University of Montreal; and Xintao Wei of UConn Health.
RNA regulation
Much of the ENCODE project has thus far relied on detecting regulatory sequences of DNA using a technique called ChIP-seq. This technique allows researchers to identify DNA sites that are bound to DNA-binding proteins such as transcription factors, helping to determine the functions of those DNA sequences.
However, Burge points out, this technique won’t detect genomic elements that must be copied into RNA before getting involved in gene regulation. Instead, the RNA team relied on a technique known as eCLIP, which uses ultraviolet light to cross-link RNA molecules with RNA-binding proteins (RBPs) inside cells. Researchers then isolate specific RBPs using antibodies and sequence the RNAs they were bound to.
RBPs have many different functions — some are splicing factors, which help to cut out sections of protein-coding messenger RNA, while others terminate transcription, enhance protein translation, break down RNA after translation, or guide RNA to a specific location in the cell. Determining the RNA sequences that are bound to RBPs can help to reveal information about the function of those RNA molecules.
“RBP binding sites are candidate functional elements in the transcriptome,” Burge says. “However, not all sites of binding have a function, so then you need to complement that with other types of assays to assess function.”
The researchers performed eCLIP on about 150 RBPs and integrated those results with data from another set of experiments in which they knocked down the expression of about 260 RBPs, one at a time, in human cells. They then measured the effects of this knockdown on the RNA molecules that interact with the protein.
Using a technique developed by Burge’s lab, the researchers were also able to narrow down more precisely where the RBPs bind to RNA. This technique, known as RNA Bind-N-Seq, reveals very short sequences, sometimes containing structural motifs such as bulges or hairpins, that RBPs bind to.
Overall, the researchers were able to study about 350 of the 1,500 known human RBPs, using one or more of these techniques per protein. RNA splicing factors often have different activity depending on where they bind in a transcript, for example activating splicing when they bind at one end of an intron and repressing it when they bind the other end. Combining the data from these techniques allowed the researchers to produce an “atlas” of maps describing how each RBP’s activity depends on its binding location.
“Why they activate in one location and repress when they bind to another location is a longstanding puzzle,” Burge says. “But having this set of maps may help researchers to figure out what protein features are associated with each pattern of activity.”
Additionally, Lecuyer’s group at the University of Montreal used green fluorescent protein to tag more than 300 RBPs and pinpoint their locations within cells, such as the nucleus, the cytoplasm, or the mitochondria. This location information can also help scientists to learn more about the functions of each RBP and the RNA it binds to.
“The strength of this manuscript is in the generation of a comprehensive and multilayered dataset that can be used by the biomedical community to develop therapies targeted to specific sites on the genome using genome-editing strategies, or on the transcriptome using antisense oligonucleotides or agents that mediate RNA interference,” says Gil Ast, a professor of human molecular genetics and biochemistry at Tel Aviv University, who was not involved in the research.
Linking RNA and disease
Many research labs around the world are now using these data in an effort to uncover links between some of the RNA sequences identified and human diseases. For many diseases, researchers have identified genetic variants called single nucleotide polymorphisms (SNPs) that are more common in people with a particular disease.
“If those occur in a protein-coding region, you can predict the effects on protein structure and function, which is done all the time. But if they occur in a noncoding region, it’s harder to figure out what they may be doing,” Burge says. “If they hit a noncoding region that we identified as binding to an RBP, and disrupt the RBP’s motif, then we could predict that the SNP may alter the splicing or stability of the gene.”
Burge and his colleagues now plan to use their RNA-based techniques to generate data on additional RNA-binding proteins.
“This work provides a resource that the human genetics community can use to help identify genetic variants that function at the RNA level,” he says.
The research was funded by the National Human Genome Research Institute ENCODE Project, as well as a grant from the Fonds de Recherche de Québec-Santé.
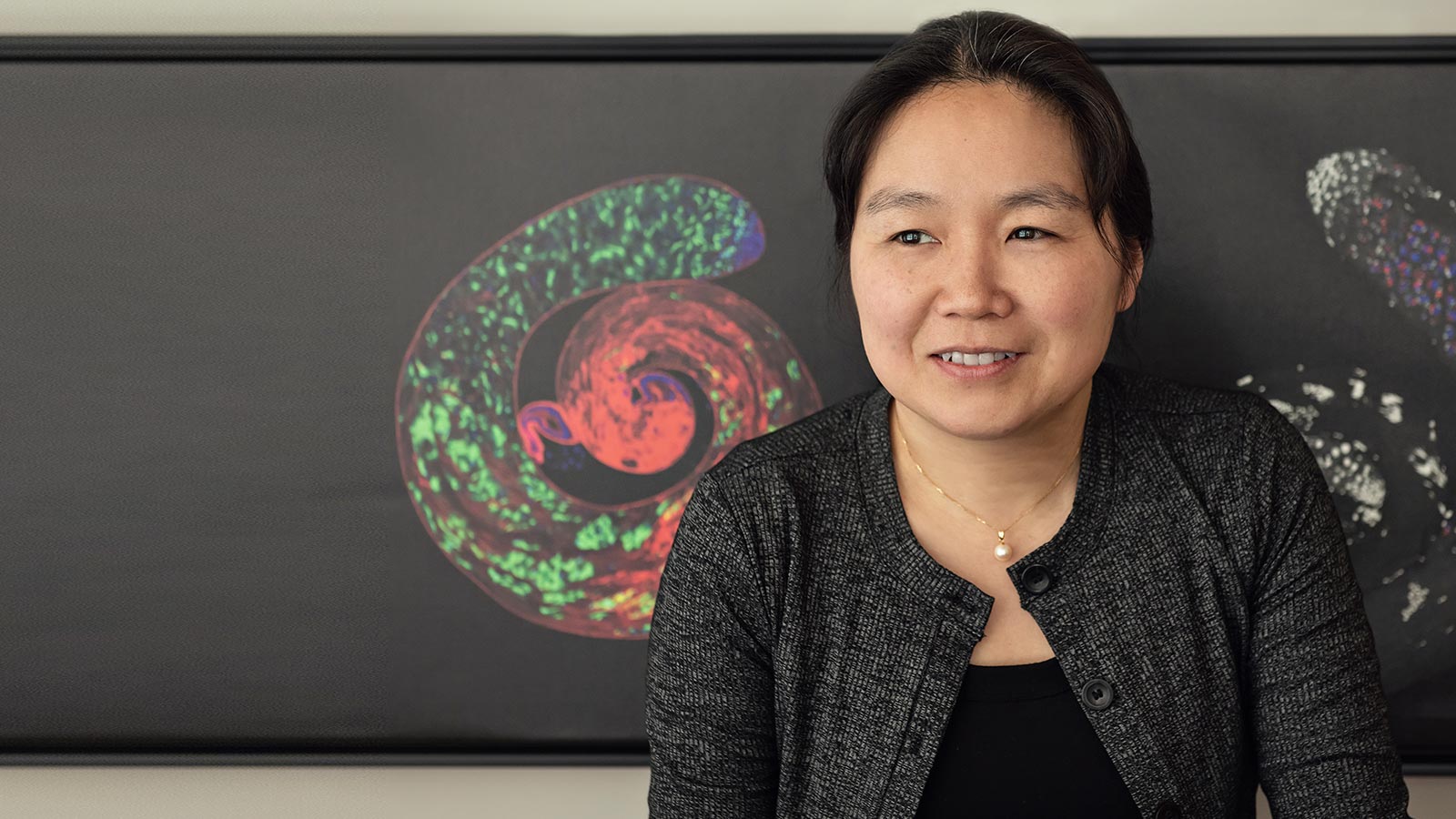
Education
- PhD, 1999, Kyoto University
- BS, Biology, 1994, Kyoto University
Research Summary
Two remarkable feats of multicellular organisms are generation of many distinct cell types via asymmetric cell division and transmission of the germline genome to the next generation, essentially in eternity. Studying these processes using the Drosophila male germline as a model system has led us to venture into new areas of study, such as functions of satellite DNA, a ‘genomic junk,’ and how they might be involved in speciation.
Awards
- National Academy of Sciences, 2025
- Tsuneko and Reiji Okazaki Award, 2016
- Howard Hughes Medical Institute, Investigator, 2014
- MacArthur Fellow, 2011
- Women in Cell Biology Early Career Award, American Society for Cell Biology, 2009
- Searle Scholar, 2008
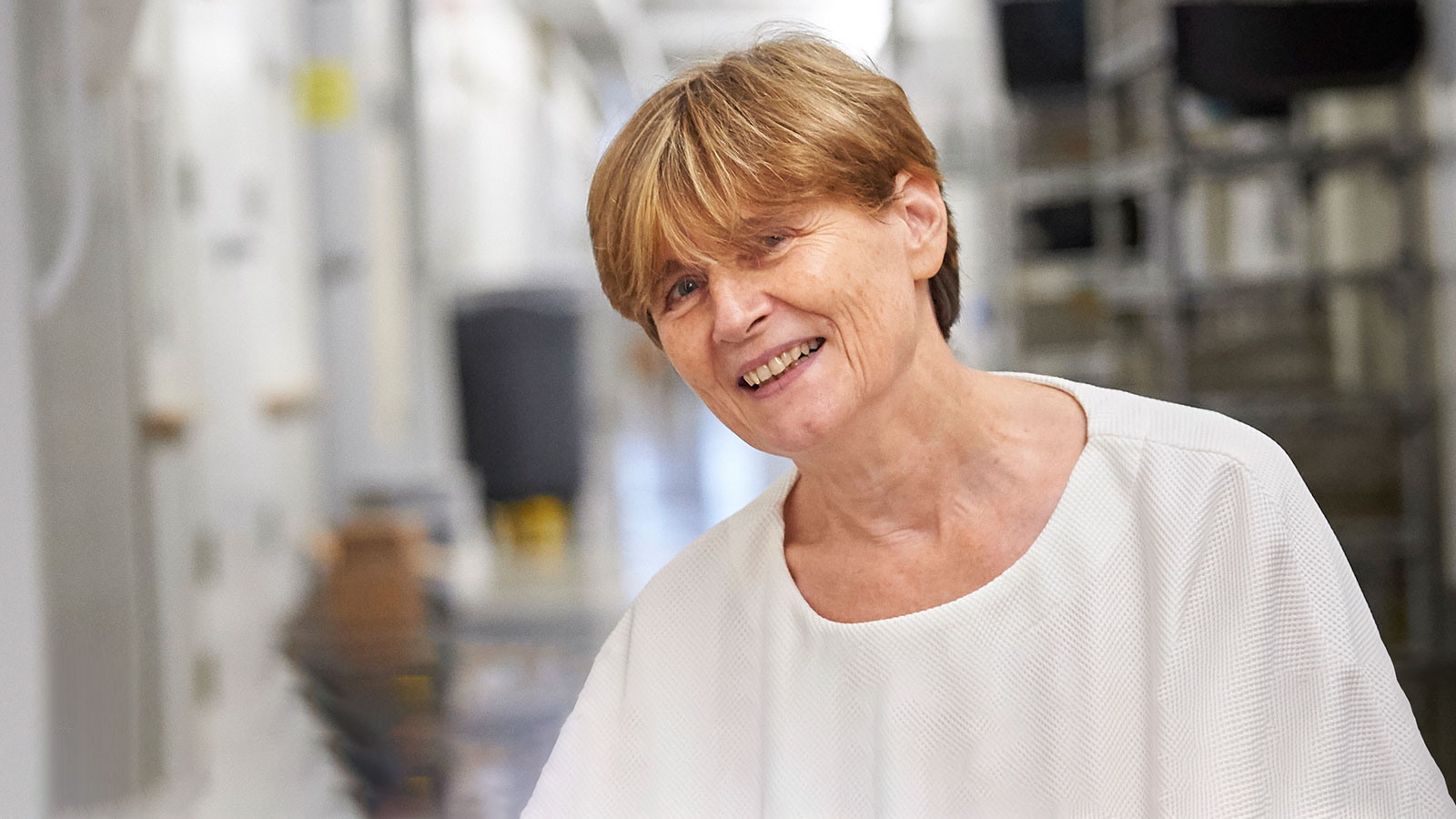
Education
- Dr. rer. nat., 1985, University of Tübingen
- MS, 1981, Biology, University of Freiburg
Research Summary
We study germ cells, the only cells in the body naturally able to generate completely new organisms. In addition to the nuclear genome, cytoplasmic information is passed though the egg cell to the next generation. We analyze the organization and regulation of germ line specific RNA-protein condensates, and explore mechanisms used by endosymbionts such as mitochondria and the intracellular bacterium, Wolbachia, to propagate through the cytoplasm of the female germ line.
Awards
- Vanderbilt Prize in Biomedical Science, 2022
- Gruber Genetics Prize, 2022
- Thomas Hunt Morgan Medal, Genetics Society of America, 2021
- Francis Amory Prize in Reproductive Medicine and Reproductive Physiology, American Academy of Arts and Sciences, 2020
- Vilcek Prize in Biomedical Science, 2020
- Keith R. Porter Award, American Society for Cell Biology, 2018
- Inaugural Klaus Sander Prize, German Society for Developmental Biology, 2017
- European Molecular Biology Organization, Foreign Associate, 2012
- Conklin Medal of the Society of Developmental Biology, 2011
- National Academy of Sciences, Foreign Associate, 2005; Member, 2008
- American Academy of Arts and Sciences, Member, 1998
- Howard Hughes Medical Institute, Investigator, 1990 and 1997