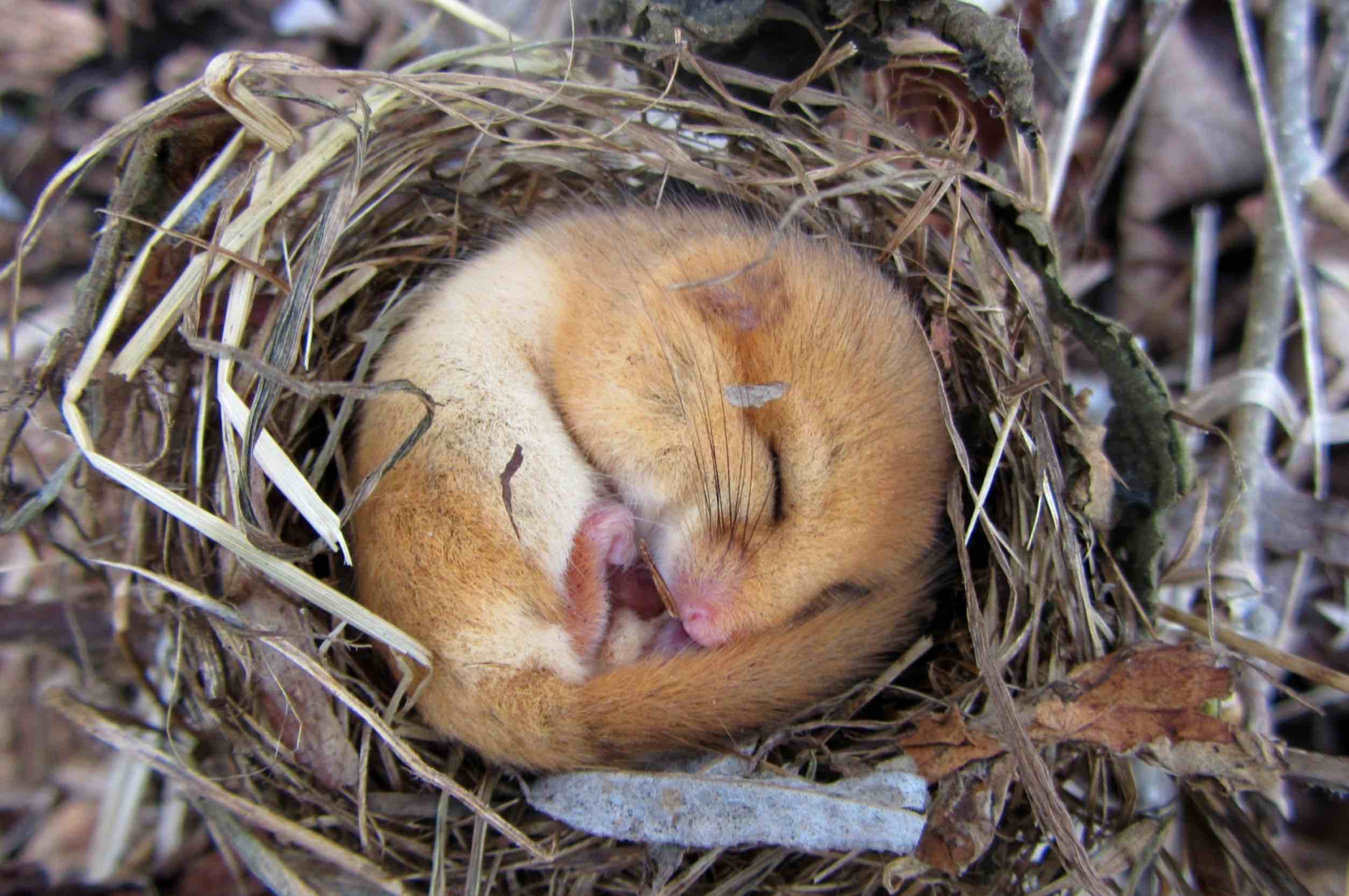
Merrill Meadow | Whitehead Institute
March 23, 2022
Metabolism is the sum of life-sustaining chemical reactions occurring in cells and across whole organisms. The human genome codes for thousands of metabolic enzymes, and specific metabolic pathways play significant roles in many biological processes—from breaking down food to release energy, to normal proliferation and differentiation of cells, to pathologies underlying diabetes, cancer, and other diseases.
For decades, Whitehead Institute researchers have helped both to clarify how metabolism works in healthy states and to identify how metabolic processes gone awry contribute to diseases. Among Whitehead Founding Member Harvey Lodish’s wide-ranging accomplishments, for example, are the identification of genes and proteins involved in development of insulin resistance and stress responses in fat cells. His lab explored the hormones controlling fatty acid and glucose metabolism, broadening understanding of obesity and type 2 diabetes. In 1995, the lab cloned adiponectin, a hormone made exclusively by fat cells. A long series of studies has shown that adiponectin causes muscle to burn fatty acids faster – so they are not stored as fat – and increases the metabolism of the sugar glucose. More recently the lab identified and characterized types of RNAs that are specifically expressed in fat cells – including a microRNA unique to brown fat, which burns rather than stores fatty acids. In addition, former Member David Sabatini’s discovery of the mTOR protein and his subsequent work elaborating many ways in which the mTOR pathway affects cells function has proven to be fundamental to understanding the relationship between metabolism and an array of diseases.
Today, Institute researchers continue to pioneer a deeper understanding of how metabolic processes contribute to health and disease – with long-term implications that could range from new treatments for obesity and type 2 diabetes to methods for slowing the aging process. Here are a few examples of Whitehead Institute scientists’ creative and pioneering work in the field of metabolism.
Understanding hibernation and torpor
Research inspiration comes in many forms. For example, Whitehead Institute Member Siniša Hrvatin – who joined the faculty in January 2022 from Harvard Medical School (HMS) – was inspired to pursue his current research by science-fiction tales about suspended animation for long-term space travel. And during graduate school, he realized that the ability of some mammals to enter a state of greatly reduced metabolism – such as occurs in hibernation – was a mild but real-world form of suspended animation.
Hrvatin’s doctoral research in Doug Melton’s lab at Harvard University focused primarily on stem cell biology. But his subsequent postdoctoral research positions at Massachusetts Institute of Technology (MIT) and HMS enabled him to begin exploring the mechanisms and impact of reduced metabolic states in mammals. The timing was serendipitous, too, because he was able to use the growing array of genetic tools that were becoming available – and create some new tools of his own as well.
“To survive extreme environments, many animals have evolved the ability to profoundly decrease metabolic rate and body temperature and enter states of dormancy, such as hibernation and torpor,” Hrvatin says. Hibernating animals enter repeated states of significantly reduced metabolic activity, each lasting days to weeks. By comparison, daily torpor is shorter, with animals entering repeated periods of lower-than-normal metabolic activity lasting several hours.
Hrvatin’s lab studies the mysteries of how animals and their cells initiate, regulate, and survive these adaptations. “Our long-term goal is to determine if these adaptations can be harnessed to create therapeutic applications for humans.” He and his team are focusing on three broad questions regarding the mechanisms underlying torpor in mice and hibernation in hamsters.
First: How do the animals’ brains initiate and regulate the metabolic processes involved in this process? During his postdoctoral research, Hrvatin published details of his discovery of neurons involved in the regulation of mouse torpor. “Now we are investigating how these torpor-regulating neurons receive information about the body’s energy-state,” he explains, “and studying how these neurons then drive a decrease of metabolic rate and body temperature throughout the body.”
Second: How do individual cells – and their genomes – adapt to extreme or changing environments; and how do these adaptations differ between types of organisms?
“Cells from hibernating organisms ranging from rodents to bears have evolved the ability to survive extreme cold temperatures for many weeks to months,” Hrvatin notes. “We are using genetic screens to identify species-specific mechanisms of tolerance to extreme cold. Then we will explore whether these mechanisms can be induced in non-hibernating organisms – potentially to provide health benefits.”
Third: Can we deliberately and specifically slow down tissue damage, disease progression, and or aging in cells and whole organisms by inducing torpor or hibernation – or facets of those states? It has long been known that hibernating animals live longer than closely related non-hibernators; that cancer cells do not replicate during hibernation; and that cold can help protect neurons from the effects of loss of oxygen. However, the cellular mechanisms underlying these phenomena remain largely unknown. Hrvatin’s lab will induce a long-term hibernation-like state in mice and natural hibernation in hamsters, and study how those states affect processes such as tissue repair, cancer progression, and aging.
“In the lab, if you take many human cell types and put them in a cold environment they die, but cells from hibernators survive,” Hrvatin notes. “We’re fascinated by the cellular processes underlying those survival capacities. As a starting place, we are using novel CRISPR screening approaches to help us identify the genomic mechanisms involved.”
And then? “Ultimately, we hope to take on the biggest question: Is it possible to transfer some of those survival abilities to humans?
Solving a mitochondrial conundrum
When Whitehead Institute postdoctoral researcher Jessica Spinelli was studying cancer metabolism in graduate school, she became interested in what seemed to be a scientific paradox regarding mitochondria, the cell’s energy-producing organelles: Mitochondria are believed to be important for tumor growth; but they generally need oxygen to function, and substantial portions of tumors have very low oxygen levels. Pursuing research in the lab of former Whitehead Institute Member David Sabatini, Spinelli sought to understand how those facts fit together and whether mitochondria could somehow adapt to function with limited oxygen levels.
Recently, Spinelli and colleagues published an answer to the conundrum – one that could inform research into medical conditions including ischemia, diabetes and cancer. In a Science paper for which Spinelli was first author, the team demonstrated that when cells are deprived of oxygen, a molecule called fumarate can serve as a substitute and enable mitochondria to continue functioning.
As Spinelli explains, humans need oxygen molecules for the cellular respiration process that takes place in our cells’ mitochondria. In this process – called the electron transport chain – electrons are passed along in a sort of cellular relay race that, ultimately, allows the cell to create the energy needed to perform its vital functions. Usually, oxygen is necessary to keep that process operating.
Using mass spectrometry to measure the quantities of molecules produced through cellular respiration in varied conditions, Spinelli and the team found that cells deprived of oxygen had a high level of succinate molecules, which form when electrons are added to a molecule called fumarate. “From this, we hypothesized that the accumulation of succinate in low-oxygen environments is caused by mitochondria using fumarate as a substitute for oxygen’s role in the electron transport chain,” Spinelli explains. “That could explain how mitochondria function with relatively little oxygen.” The next step was to test that hypothesis in mice, and those studies provided several interesting findings: Only mitochondria in kidney, liver, and brain tissues could use fumarate in the electron transport chain. And even in normal conditions, mitochondria in these tissues used both fumarate and oxygen to function – shifting to rely more heavily on fumarate when oxygen was reduced. In contrast, heart and skeletal muscle mitochondria made minimal use of fumarate and did not function well with limited oxygen.
“We foresee some exciting work ahead, learning exactly how this process is regulated in different tissues,” Spinelli says, “and, especially, in solid tumor cancers, where oxygen levels vary between regions.”
Seeking a more accurate model of diabetes
Max Friesen, a postdoctoral researcher in the lab of Whitehead Institute Founding Member Rudolf Jaenisch, studies the role of cell metabolism in type 2 diabetes (T2D). An increasingly prevalent disease that affects millions of people around the world, T2D is hard to study in the lab. This has made it very challenging for scientists to detail the cellular mechanisms through which it develops – and therefore to create effective therapeutics.
“It has always been very hard to model T2D, because metabolism differs greatly between species,” Friesen says. “That fact leads to complications when we use animal models to study this disease. Mice, for example, have much higher metabolism and faster heart rates than humans. As a result, researchers have developed many approaches that cure diabetes in mice but that fail in humans.” Nor do most in vitro culture systems—cells in a dish—effectively recapitulate the disease.
But, building on Jaenisch’s pioneering success in developing disease models derived from human stem cells, Friesen is working to create a much more accurate in vitro system for studying diabetes. His goal is to make human stem cell-derived tissues that function as they would in the human body, closely recapitulating what happens when an individual develops diabetes. Currently, Friesen is differentiating human stem cells into metabolic tissues such as liver and adipocytes (fat). He has improved current differentiation protocols by adapting these cells to a culture medium that is much closer to the environment they see in the human body. Serendipitously, the process also makes the cells responsive to insulin at levels that are present in the human bloodstream. “This serves as a great model of a healthy cell that we can then turn into a disease model by exposing the cell to diabetic hyperinsulinemia,” Friesen says.
These advances should enable him to gain a better understanding of how metabolic pathways – such as the insulin signaling pathway – function in a diabetic model versus a healthy control model. “My hope is that our new models will enable us to figure out how dietary insulin resistance develops, and then identify a therapeutic intervention that blocks that disease-causing process,” he explains. “It would be fantastic to help alleviate this growing global health burden.”