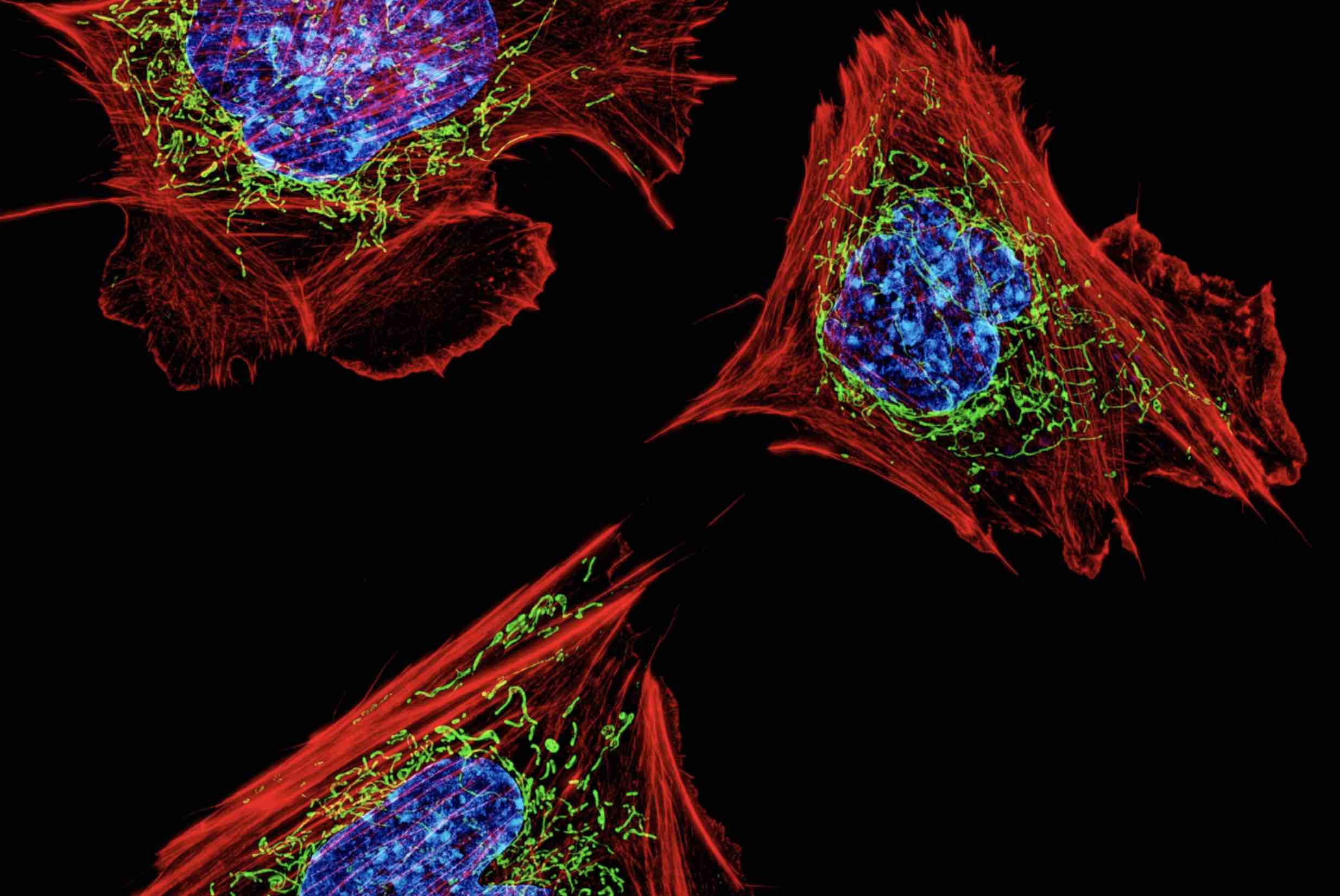
Eva Frederick | Whitehead Institute
December 2, 2021
Humans need oxygen molecules for a process called cellular respiration, which takes place in our cells’ mitochondria. Through a series of reactions called the electron transport chain, electrons are passed along in a sort of cellular relay race, allowing the cell to create ATP, the molecule that gives our cells energy to complete their vital functions.
At the end of this chain, two electrons remain, which are typically passed off to oxygen, the “terminal electron acceptor.” This completes the reaction and allows the process to continue with more electrons entering the electron transport chain.
In the past, however, scientists have noticed that cells are able to maintain some functions of the electron transport chain, even in the absence of oxygen. “This indicated that mitochondria could actually have partial function, even when oxygen is not the electron acceptor,” said Whitehead Institute postdoctoral researcher Jessica Spinelli. “We wanted to understand, how does this work? How are mitochondria capable of maintaining these electron inputs when oxygen is not the terminal electron acceptor?”
In a paper published December 2 in the journal Science, Whitehead Institute scientists and collaborators led by Spinelli have found the answer to these questions. Their research shows that when cells are deprived of oxygen, another molecule called fumarate can step in and serve as a terminal electron acceptor to enable mitochondrial function in this environment. The research, which was completed in the laboratory of former Whitehead Member David Sabatini, answers a long-standing mystery in the field of cellular metabolism, and could potentially inform research into diseases that cause low oxygen levels in tissues, including ischemia, diabetes and cancer.
A new runner in the cellular relay
The researchers began their investigation into how cells can maintain mitochondrial function without oxygen by using mass spectrometry to measure the quantities of molecules called metabolites that are produced through cellular respiration in both normal and low-oxygen conditions. When cells were deprived of oxygen, researchers noticed a high level of a molecule called succinate.
When you add electrons to oxygen at the end of the electron transport chain, it picks up two protons and becomes water. When you add electrons to fumarate, it becomes succinate. “This led us to think that maybe this accumulation of succinate that’s occurring could actually be caused by fumarate being used as an electron acceptor, and that this reaction could explain the maintenance of mitochondrial functions in hypoxia,” Spinelli said.
Usually, the fumarate-succinate reaction runs the other direction in cells — a protein complex called the SDH complex takes away electrons from succinate, leaving fumarate. For the opposite to happen, the SDH complex would need to be running in reverse. “Although the SDH complex is known to catalyze some fumarate reduction, it was thought that it was thermodynamically impossible for this SDH complex to undergo a net reversal,” Spinelli said. “Fumarate is used as an electron acceptor in lower eukaryotes, but they use a totally different enzyme and electron carrier, and mammals are not known to possess either of these.”
Through a series of assays, however, the researchers were able to ascertain that this complex was indeed running in reverse in cultured cells, largely due to accumulation of a molecule called ubiquinol, which the researchers observed to build up under low-oxygen conditions.
With their hypothesis confirmed, “We wanted to get back to our initial question and ask, does that net reversal of the SDH complex maintain mitochondrial functions which are happening when cells are exposed to hypoxia?” said Spinelli. “So, we knocked out SDH complex and then we demonstrated through a number of means that loss of both oxygen and fumarate as an electron acceptors was sufficient to [bring the electron transport chain to a halt].”
All this work was done in cultured cells, so the next step for Spinelli and collaborators was to study whether fumarate could serve as a terminal electron acceptor in mouse models.
When they tried this, the team uncovered something interesting: some, but not all, of the mice’s tissues were able to successfully reverse the activity of the SDH complex and perform mitochondrial functions using fumarate as a terminal electron acceptor.
“What was really cool to see is that there were three tissues — the kidney, the liver, and the brain — which on a bulk tissue scale, are operating the SDH complex in a backwards direction, even at physiological oxygen levels,” said Spinelli. In other words, even in normal conditions, these tissues were reducing both fumarate and oxygen to maintain their functions, and when deprived of oxygen, fumarate could take over as a terminal electron acceptor.
In contrast, tissues such as the heart and the skeletal muscle are able to perform minimal fumarate reduction without reversing the SDH complex, but not to the extent that they could effectively retain mitochondrial function when deprived of oxygen.
“We think there’s a lot of exciting work downstream of this to figure out how exactly this process is regulated differently in different tissues — and understanding in disease settings whether the SDH complex is operating in the net reverse direction,” Spinelli said.
In particular, Spinelli is interested in studying the behavior of the SDH complex in cancer cells.
“Certain regions of solid tumors have very low levels of oxygen, and certain regions have high levels of oxygen,” Spinelli said. “It’s interesting to think about how those cells are surviving in that microenvironment — are they using fumarate as an electron acceptor to enable cell survival?”