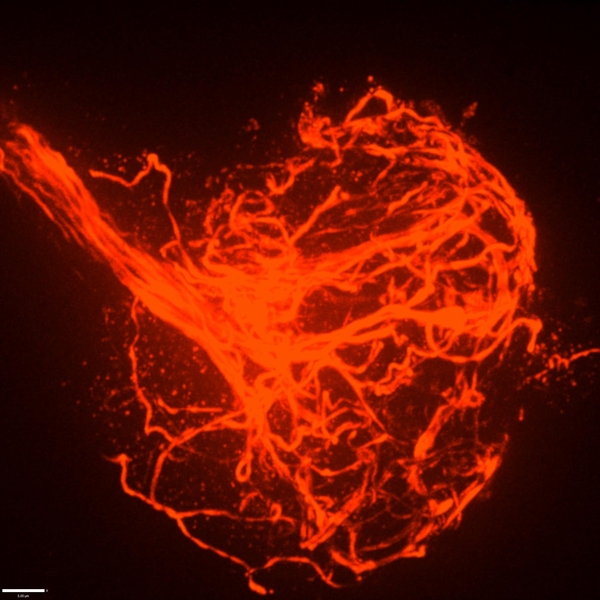
David Orenstein | The Picower Institute for Learning and Memory
June 23, 2023
Perhaps the most obvious feature of a neuron is the long branch called an axon that ventures far from the cell body to connect with other neurons or muscles. If that long, thin projection ever seems like it could be vulnerable, a new MIT study shows that its structural integrity may indeed require the support of a surrounding protein called perlecan. Without that protein in Drosophila fruit flies, researchers at The Picower Institute for Learning and Memory found axonal segments can break apart during development and the connections, or synapses, that they form end up dying away.
Perlecan helps make the extracellular matrix, the proteins and other molecules that surround cells, stable and flexible so that cells can develop and function in an environment that is supportive without being rigid.
“What we found was that the extracellular matrix around nerves was being altered and essentially causing the nerves to break completely. Broken nerves eventually led to the synapses retracting,” says study senior author Troy Littleton, the Menicon Professor in MIT’s departments of Biology and Brain and Cognitive Sciences.
Humans need at least some perlecan to survive after birth. Mutations that reduce, but don’t eliminate, perlecan can cause Schwartz-Jampel syndrome, in which patients experience neuromuscular problems and skeletal abnormalities. The new study may help explain how neurons are affected in the condition, Littleton says, and also deepen scientists’ understanding of how the extracellular matrix supports axon and neural circuit development.
Ellen Guss PhD ’23, who recently defended her doctoral thesis on the work, led the research published June 8 in eLife.
At first she and Littleton didn’t expect the study to yield a new discovery about the durability of developing axons. Instead, they were investigating a hypothesis that perlecan might help organize some of the protein components in synapses that fly nerves develop to connect with muscles. But when they knocked out the gene called “trol” that encodes perlecan in flies, they saw that the neurons appeared to “retract” many synapses at a late stage of larval development. Proteins on the muscle side of the synaptic connection remained, but the neuron side of the connection withered away. That suggested that perlecan had a bigger role than they first thought.
Indeed, the authors found that the perlecan wasn’t particularly enriched around synapses. Where it was pronounced was in a structure called the neural lamella, which surrounds axon bundles and acts a bit like the rubbery cladding around a TV cable to keep the structure intact. That suggested that a lack of perlecan might not be a problem at the synapse, but instead causes trouble along axons due to its absence in the extracellular matrix surrounding nerve bundles.
Littleton’s lab had developed a technique for daily imaging of fly neural development called serial intravital imaging. They applied it to watch what happened to the fly axons and synapses over a four-day span. They observed that while fly axons and synapses developed normally at first, not only synapses but also whole segments of axons faded away.
They also saw that the farther an axon segment was from the fly’s brain, the more likely it was to break apart, suggesting that the axon segments became more vulnerable the further out they extended. Looking segment by segment, they found that where axons were breaking down, synapse loss would soon follow, suggesting that axon breakage was the cause of the synapse retraction.
“The breakages were happening in a segment-wide manner,” Littleton says. “In some segments the nerves would break and in some they wouldn’t. Whenever there was a breakage event, you would see all the neuromuscular junctions (synapses) across all the muscles in that segment retract.”
When they compared the structure of the lamella in mutant versus healthy flies, they found that the lamella was thinner and defective in the mutants. Moreover, where the lamella was weakened, axons were prone to break and the microtubule structures that run the length of the axon would become misdirected, protruding outward and becoming tangled up in dramatic bundles at sites of severed axons.
In one other key finding, the team showed that perlecan’s critical role depended on its secretion from many cells, not just neurons. Blocking the protein in just one cell type or another did not cause the problems that total knockdown did, and enhancing secretion from just neurons was not enough to overcome its deficiency from other sources.
Altogether, the evidence pointed to a scenario where lack of perlecan secretion caused the neural lamella to be thin and defective, with the extracellular matrix becoming too rigid. The further from the brain nerve bundles extended, the more likely movement stresses would cause the axons to break where the lamella had broken down. The microtubule structure within the axons then became disorganized. That ultimately led to synapses downstream of those breakages dying away because the disruption of the microtubules means the cells could no longer support the synapses.
“When you don’t have that flexibility, although the extracellular matrix is still there, it becomes very rigid and tight and that basically leads to this breakage as the animal moves and pulls on those nerves over time,” Littleton says. “It argues that the extracellular matrix is functional early on and can support development, but doesn’t have the right properties to sustain some key functions over time as the animal begins to move and navigate around. The loss of flexibility becomes really critical.”
In addition to Littleton and Guss, the paper’s other authors are Yulia Akbergenova and Karen Cunningham.
Support for the study came from the National Institutes of Health. The Littleton Lab is also supported by The Picower Institute for Learning and Memory and The JPB Foundation.